1. INTRODUCTION
Diabetes mellitus (DM) is among the most common chronic metabolic disorders, and is characterized by hyperglycemia due to a lack of insulin or perturbations in insulin signaling [1, 2]. Diabetes has become a worldwide public health crisis. Owing to the aging of populations worldwide, the prevalence of diabetes and pre-diabetes has been predicted to significantly increase in the coming decades [3].
Type 1 and type 2 diabetes both exist pancreas dysfunction, therefore, it is hard for β-cells to keep up with the body’s increased need for insulin [4]. A family history of diabetes is considered a major risk factor for both T2DM and pre-diabetes [5, 6]. Currently, patients with diabetes are advised to adhere to an appropriate diet with restricted energy intake or to lifelong use of hypoglycemic drugs [7]. Needle free injection and insulin pumps provide treatment flexibility but are not curative. Patients with diabetes have a mortality rate due to cardiovascular disease that is two to four times higher than that of unaffected individuals, because of the incidence of heart failure [8]. Therefore, a better understanding of the mechanism of diabetic cardiomyopathy will aid in developing novel strategies for patients with DM.
Abnormal glycolipid metabolism is closely associated with diabetes, atherosclerosis, coronary disease, hypertriglyceridemia, low high-density lipoprotein (HDL) cholesterolemia, insulin resistance (IR) and other diseases. Free radicals, including reactive oxygen species (ROS) and nitric oxide, are highly reactive byproducts of oxidation and biochemical reactions in human cells. In recent years, the antioxidant activity of naturally occurring substances in higher plants and cells has been reported. Carotenoids are natural lipophilic pigments synthesized by plants, algae, fungi and bacteria [9]. Most carotenoids are lipophilic and always associated with bio-membranes, so that they have great potential to cross the blood-brain barrier [10]. Their high biological activity is known to confer various health advantages. Because of their chemical structure and interaction with biological membranes, carotenoids have potential biological antioxidant properties [11]. More than 600 types of carotenoids have been identified in nature. However, according to molecular structure, only α-carotene, β-carotene, lycopene and xanthophylls are usually present in the human diet [12].
β-carotene is highly abundant in carotenoids and is an important food coloring agent [13]. The absorption or accumulation of β-carotene varies among species. To date, the effect of β-carotene on glycolipid metabolism remains unclear. Little is known regarding β-carotene metabolic turnover or pathways in humans. Adequate nutritional intake is a non-pharmacological intervention and prevention strategy. Long-term high blood glucose levels may induce cardiomyocyte apoptosis, cardiac dysfunction and fetal cardiomyocyte proliferation. Therefore, we examined the hypoglycemic effects of β-carotene in a type 2 diabetes mellitus (T2DM) model and assessed its protective effects in rat cardiomyocytes.
2. METHODS
2.1 Study participants
We obtained publicly available data from the NHANES website: https://wwwn.cdc.gov/nchs/nhanes/ Our study population comprised participants in the 2001–2006 cycles who were examined with NCHS recommended methods [14]. NHANES selected participants through stratified, multistage, clustered probability sampling. Our analysis included participants (1 year of age and older) who had biological measurements for both serum cis-β-carotene and fasting glucose. The final sample size of 9281 individuals was tested for the covariates included in the model. Participants were excluded if their data were incomplete or invalid. Missing values in data can decrease model power and lead to incorrect predictions and classifications. Therefore, we used generalized mean imputation methods to replace missing values. Linear regression analysis was used to illustrate the correlation between fasting blood glucose (FBG) and serum levels of cis-β-carotene.
2.2 Laboratory assessments
Blood analysis data were obtained from participants randomly assigned to receive examination in the morning after an overnight fast. Low-density lipoprotein (LDL) cholesterol, triglycerides (TG), C-peptide, C-reactive protein and fasting glucose were assessed according to the NHANES laboratory protocol.
2.3 Animals, diets and treatments
An overview of the proposed workflow is shown in Figure 1 . We used 6- to 7-week-old healthy male Sprague–Dawley rats (n=30, weighing 200±30 g) kept in our institutional animal facility. All rats were acclimated and housed under standard laboratory conditions (12-h light/dark cycle at 10–30% humidity and 22–26°C), and were given free access to tap water and food. All experiments were performed at the same time in the morning to minimize differences in circadian rhythms. All animal studies were conducted in accordance with the guidelines for the Care and Use of Laboratory Animals. Rats were designed and randomly divided into a control group (n=10) receiving a standard diet (overall calories: 3.5 kcal/g) and streptozotocin-induced-diabetes group (DM, n=20). To mimic the features of T2DM in humans, we injected DM rats intra-peritoneally with low-dose streptozotocin at 20 mg/kg body weight for 3 days. After an additional week, the rats with FBG >16.7 mmol/L were included in the study as T2DM rats [15], which were fed a high-fat diet containing 20% protein, 60% fat, 20% carbohydrate and 60% fat (D12492, Research Diets Inc.) for 8 weeks. DM rats were further divided into 4-week (DM and DM+β-carotene) and 8-week (DM and DM+β-carotene) subgroups. The β-carotene group received oral supplementation of β-carotene (50 mg/kg) by gavage.
2.4 Echocardiography measurements
Cardiac function in rats was assessed with echocardiography, which was performed on self-breathing rats under anesthesia through intraperitoneal injection of 10% chloral hydrate according to body weight, as reported previously [16]. LV end diastolic/systolic diameters (LVEDD and LVESD) and LV posterior wall thickness in diastole and systole (LVPWd and LVPWs) were measured in M-mode recordings. Values of all echocardiography measurements were averaged for at least three consecutive cardiac cycles.
Contrast-enhanced ultrasound of cardiac tissue was performed before and after the end of the insulin infusion to obtain cardiac MBV and microvascular blood-flow velocity (MFV). Microvascular blood flow (MBF) was the product of microvascular blood volume (MBV) and MFV. After the blood micro bubble concentrations reached steady state (within 2–3 min), intermittent ultra-harmonic imaging was performed with pulsing intervals of 1, 8, 12, 16, 20 and 32 cardiac cycles in the cardiac muscle. At least three images were captured digitally at each pulsing interval. Micro bubbles are small round structures with inserted gas-filled, as a contrast agent, to trace the microvasculature and provide data about MBF. Cardiac imaging included the apical two-, three-, and four-chamber views. Animals were killed with an overdose of sodium pentobarbital (100 mg/kg), and cardiac tissues were collected for biochemical and morphological studies.
2.5 Ethics approval and consent
The NHANES 1998–2012 protocol was approved by the Institutional Review Board of the Centers for Disease Control and Prevention (https://www.cdc.gov/nchs/nhanes/irba98.htm). Written informed consent were obtained from the parents of the children and from adult participants. Animal experiments were approved by the Ethical Committee for Animal Experiments at the Second Affiliated Hospital of Harbin Medical University (approval number SYDW2019-50).
2.6 Chemicals and reagents
A rat insulin ELISA kit (No. RAB0904) and streptozotocin (No. S0130) were obtained from Sigma (St. Louis). A blood glucose meter and test strips (Roche Diagnostics) were used to measure the FBG of each group once per week during the experiment.
2.7 Induction of the model of insulin-resistant primary cardiomyocytes and glucose uptake assays
For palmitic acid (PA)-induced IR experiments, a stock solution of 100 mM PA with 100 mM NaOH at 70°C in 10% (w/v) BSA was prepared to obtain solutions with various concentrations of PA [17]. Different concentrations of PA (0, 150, 250 or 350 μM) were added to primary cardiomyocytes and incubated for 24 h, and cellular glucose uptake was measured with 2-[N-(7-nitobenz-2-oxa-1,3-diazol-4-yl)amino]-2-deoxy-d-glucose (2-NBDG) (Sigma-Aldrich) at excitation and emission wavelengths of 485 and 535 nm, respectively. Briefly, cells were incubated with PA in the presence of β-carotene or metformin (as a positive control) for 24 h. Cells were seeded in glucose-free Dulbecco’s modified Eagle’s medium for 4 h, then stimulated with insulin (500 nM) for 10 min. After incubation with 50 nM 2-NBDG in glucose-free Dulbecco’s modified Eagle’s medium, the cells were gently washed with cold PBS buffer to remove the 2-NBDG.
2.8 Quantitative reverse-transcription polymerase chain reaction
Total RNA was extracted with TRIzol (Life Technologies, Carlsbad) according to the manufacturer’s instructions, and reverse transcribed into cDNA with reverse transcriptase. The primer sequences were as follows: PGC-1β: forward AACCCAACCAGTCTCACA, reverse CTCCTAGGGGCCTTTGTT; TFAM: forward CCAAAAAGACCTCGTTCA, reverse ATGTCTCCGGATCG TTTC; and NRF1: forward TATGGCGGAAGTAATGAA, reverse CAACGTAAGCTCTGCC.
2.9 Biochemical analysis
A GLUT4 BioAssay ELISA kit (US Biological, No. 383107), Total IRS-1 Cell-Based Colorimetric ELISA kit (Immunoway, No. KA4145C) and Akt (pS473)+total Akt ELISA kit (Abcam, No. ab126433) were used according to the manufacturer’s instructions. The protocols of the kits were all relatively simple. All prepared samples were pipetted into the wells, and samples were bound to the wells by immobilized antibodies. The stop solution changed the color from the original blue to yellow, and the absorbance was read with a microplate reader at 450 nm with an optional reference wavelength of 665 nm.
2.10 Statistical analysis
Our data are reported as the mean ± standard error of the mean (SEM) for at least three independent experimental replicates. One-way ANOVA followed by Tukey’s post hoc test was performed to assess significance in three or more groups. Two-way ANOVA with Holm-Sidak test was used for performance comparisons in Graph Pad Prism 7. The threshold for significant differences was P<0.05.
3. RESULTS
3.1 Demographic and baseline characteristic data
In total, data for 31509 NHANES participants (2001–2006) were collected; 9281 participants had complete blood glucose and cis-β-carotene data. The participants were categorized into four groups on the basis of serum cis-β-carotene quartiles ( Table 1 ). A total of 9281 participants (4557 males and 4724 females) 38.82±22.28 years of age (range 0–85 years) with a body mass index (BMI) of 27.07±6.72 kg/m2 were included in this study. Compared with the other groups, the Q4 group was older (Q4, 49.0±22.8 vs. Q3, 38.3±22.6 vs. Q2, 33.6±20.0, P<0.001), and had a higher systolic blood pressure (SBP) (Q4, 124.09±23.20 vs. Q3, 119.33±19.90 vs. Q2, 118.01±17.74, P<0.001) and a higher ratio of family income to poverty threshold. Participants with high levels of cis-β-carotene had lower FBG, fasting insulin, homeostatic model assessment of insulin resistance (HOMA-IR) and Homeostasis model assessment-β (HOMA-B). Notably, cis-β-carotene levels were negatively associated with FBG and insulin levels.
Baseline socio-demographic and clinical characteristics of the study population according to serum cis-β-carotene quartiles
Characteristic | Cis-β-carotene (0.004–0.402 μmol/L) | P value | |||
---|---|---|---|---|---|
Quartile 1 | Quartile 2 | Quartile 3 | Quartile 4 | ||
Participants, n | 624 | 3845 | 2407 | 2405 | |
Age, years | 33.4±18.8 | 33.6±20.0 | 38.3±22.6 | 49.0±22.8 | <0.001 |
Sex | |||||
Male | 373 (59.8%) | 2063 (53.7%) | 1197 (49.7%) | 924 (38.4%) | |
Female | 251 (40.2%) | 1782 (46.3%) | 1210 (50.3%) | 1481 (61.6%) | |
BMI (kg/m2) | 28.49±6.84 | 27.86±7.42 | 26.52±6.35 | 25.97±5.39 | <0.001 |
Systolic blood pressure (mm Hg) | 118.96±17.07 | 118.01±17.74 | 119.33±19.90 | 124.09±23.20 | <0.001 |
Diastolic blood pressure (mm Hg) | 66.73±13.80 | 67.40±13.61 | 66.58±13.54 | 67.85±14.16 | 0.108 |
Ratio of family income to poverty threshold | 2.10±1.52 | 2.36±1.57 | 2.46±1.61 | 2.84±1.64 | <0.001 |
High-school education or higher, n (%) | 389 (62.3%) | 2516 (65.43%) | 1574 (65.39%) | 1735 (72.14%) | <0.001 |
Waist circumference (cm) | 97.26±17.91 | 94.34±18.14 | 91.40±16.71 | 90.70±14.60 | <0.001 |
Thigh circumference (cm) | 54.43±8.13 | 53.61±7.82 | 51.90±7.22 | 50.83±6.57 | <0.001 |
FBG (mmol/L) | 5.71±0.44 | 5.64±1.78 | 5.60±1.82 | 5.54±1.57 | 0.046 |
Fasting insulin (pmol/L) | 90.43±103.70 | 90.76±98.18 | 73.31±75.91 | 59.84±72.23 | <0.001 |
C-peptide (mmol/L) | 0.95±0.54 | 0.85±0.46 | 0.79±0. 45 | 0.72±0.37 | <0.001 |
C-reactive protein (mg/dL) | 0.55±1.31 | 0.44±0.80 | 0.38±0.78 | 0.34±0.84 | <0.001 |
TG (mmol/L) | 1.67±2.13 | 1.50±1.29 | 1.46±1.12 | 1.41±1.22 | <0.001 |
LDL-C (mmol/L) | 2.57±0.99 | 2.67±0.85 | 2.86±0.90 | 3.14±0.99 | <0.001 |
HOMA-IR | 3.48±4.88 | 3.47±5.03 | 2.86±4.65 | 2.28±3.60 | <0.001 |
HOMA-B | 170.87±742.09 | 138.63±73.84 | 118.98±218.06 | 90.45±245.77 | <0.001 |
α-Carotene (μmol/L) | 0.02±0.02 | 0.03±0.03 | 0.06±0.04 | 0.15±0.17 | <0.001 |
Trans-β-carotene (μmol/L) | 0.08±0.04 | 0.14±0.06 | 0.26±0.08 | 0.67±0.50 | <0.001 |
Combined lutein/zeaxanthin (μmol/L) | 0.20±0.08 | 0.22±0.09 | 0.27±0.12 | 0.37±0.18 | <0.001 |
Trans-lycopene (μmol/L) | 0.33±0.18 | 0.40±0.19 | 0.43±0.20 | 0.45±0.22 | <0.001 |
Note: HOMA-IR was calculated with the formula: fasting insulin (pmol/l)×FPG (mmol/l)/156.3. Insulin resistance was defined as HOMA-IR≥2.5 [23]. HOMA-B was calculated according to the formula (2.9×fasting insulin [pmol/l])/(FPG [mmol/l-3.5)]. Values are mean±SD or n (%)
3.2 Relationships among variables in the present study
We performed a correlation analysis with the Pearson correlation coefficient to determine the strength of the relationship between cis-β-carotene and blood biochemistry parameters in 9281 participants ( Table 2 ). FBG, TG, insulin and HOMA-R were negatively correlated with cis-β-carotene (P<0.001, r=-0.034; P<0.001, r=-0.041; P<0.001, r=-0.113; P<0.001, r=-0.088). The average SBP positively correlated with cis-β-carotene, FBG, TG, insulin and HOMA-R (P<0.001, r=0.109; P<0.001, r=0.220; P<0.001, r=0.145; P<0.001, r=0.331; P<0.001, r=0.094). HOMA-R was negatively correlated with cis-β-carotene (p<0.001, r=-0.088), and positively correlated with FBG, TG, insulin and SBP (P<0.001, r=0.481; P<0.001, r=0.198; P<0.001, r=0.895; P<0.001, r=0.094) according to Pearson correlation analysis.
Correlation between cis-β-carotene in blood biochemistry
Variables | Cis-β-carotene (μmol/L) | FBG (mmol/L) | TG (mmol/L) | Insulin (pmol/L) | SBP average | DBP average | HOMA-R | |||||||
---|---|---|---|---|---|---|---|---|---|---|---|---|---|---|
r value | P value | r value | P value | r value | P value | r value | P value | r value | P value | r value | P value | r value | P value | |
Cis-β-carotene (μmol/L) | 1 | -0.034 | <0.001 | -0.041 | <0.001 | -0.113 | <0.001 | 0.109 | <0.001 | -0.003 | 0.831 | -0.088 | <0.001 | |
FBG (mmol/L) | -0.034 | 0.339 | 1 | 0.240 | <0.001 | 0.220 | <0.001 | 0.220 | <0.001 | 0.072 | <0.001 | 0.481 | <0.001 | |
TG (mmol/L) | -0.041 | <0.001 | 0.240 | <0.001 | 1 | 0.174 | <0.001 | 0.145 | <0.001 | 0.098 | <0.001 | 0.198 | <0.001 | |
Insulin: SI (pmol/L) | -0.113 | <0.001 | 0.220 | <0.001 | 0.174 | <0.001 | 1 | 0.045 | <0.001 | -0.008 | <0.001 | 0.895 | <0.001 | |
SBP average | 0.109 | <0.001 | 0.22 | <0.001 | 0.145 | <0.001 | 0.045 | <0.001 | 1 | 0.331 | <0.001 | 0.094 | <0.001 | |
DBP average | -0.003 | 0.831 | 0.072 | <0.001 | 0.098 | <0.001 | -0.008 | 0.511 | 0.331 | <0.001 | 1 | 0.005 | 0.679 | |
HOMA-R | -0.088 | <0.001 | 0.481 | <0.001 | 0.198 | <0.001 | 0.895 | <0.001 | 0.094 | <0.001 | 0.005 | 0.679 | 1 |
Notes: Pearson correlation was calculated to assess the strength of the relationship between cis-β-carotene and blood biochemistry.
3.3 Associations between FBG and serum cis-β-carotene
Multivariate logistic regression analysis was used to determine the association between serum cis-β-carotene and FBG. Serum cis-β-carotene was significantly associated with FBG after multivariate adjustment for age, sex, ratio of family income to the poverty threshold, BMI, diastolic blood pressure, systolic blood pressure, LDL-cholesterol (LDL-C) and C-reactive protein ( Table 3 ). Every 1 μmol/L increase in serum cis-β-carotene levels was associated with a 0.013 mmol/L (P<0.001, 95% CI=0.009 to 0.017) and -8.01 (P<0.001, 95% CI=-12.64 to -3.56) decrease in FBG and HOMA-IR, respectively.
Association between serum fasting glucose (mmol/L) and cis-β-carotene (μmol/L)
Per 1 μmol/L increased in serum cis-β-carotene | Model 1 β (95% CI) P value | Model 2 β (95% CI) P value | Model 3 β (95% CI) P value |
---|---|---|---|
Participants | 9281 | 8537 | 5742 |
Fasting glucose (mmol/L) | 0.10 (0.009,0.011) <0.001 | 0.001 (-0.001,0.003) 0.537 | 0.013 (0.009, 0.017) <0.001 |
HOMA-IR | 0.022 (0.021,0.022) <0.001 | 0.005 (0.003, 0.007) <0.001 | 0.017 (0.014, 0.021) <0.001 |
HOMA-B | 0.022 (0.021,0.022) <0.001 | 0.004 (0.002, 0.006) <0.001 | 0.013 (0.009, 0.017) <0.001 |
TG (mmol) | 0.010 (0.009,0.011) <0.001 | 0.04 (0.003, 0.006) <0.001 | 0.013 (0.009, 0.017) <0.001 |
Insulin (pmol/L) | 0.023 (0.022,0.024) <0.001 | 0.006 (0.004, 0.008) <0.001 | 0.009 (0.006, 0.012) <0.001 |
Notes: Model 1 adjusted for no covariates. Model 2 adjusted for age, sex and the ratio of family income to the poverty threshold. Model 3 adjusted for systolic blood pressure, diastolic blood pressure, BMI, LDL-C and C-reactive protein. The subgroup analysis was stratified by sex and educational level.
3.4 Subgroup analyses of the associations between cis-β-carotene and glucose metabolism in participants
According to Table 3 , we summarized the results of subgroup analyses for adults, as shown in Figure 2 . Participants with female sex and HOMA-IR<2.5 were likely to have lower plasma glucose levels and a trend toward higher insulin levels ( Figure 3A–C ). Cis-β-carotene had a positive effect on glycemic control as well as measures of insulin sensitivity. To extend these results, we explored whether the presence of cis-β-carotene might influence glucose metabolism in the human body.
3.5 Establishment of a T2DM rat model
The elevation in blood glucose levels with respect to the control-group levels became more pronounced at 4 and 8 weeks (*P<0.05), thus indicating that the T2DM model had been successfully established [15]. Although no statistically significant changes in FBG, food intake or water intake were observed, the dynamic monitoring of β-carotene treated diabetic rats revealed a tendency toward decreased food intake during the 8-week treatment ( Figure 3A, B ).

Blood glucose levels, and food and water intake in rats.
(A). Blood glucose levels (mg/dl) obtained via tail vein puncture during the study period at 4- to 8-week intervals. (B) Food and (C) water intake in the diabetes (DM) and DM+β-carotene groups. Values are given as mean±SEM; n=10.
SOD and GSH-Px, are endogenous molecules to limit over accumulation of ROS in the body protect cells from high glucose induced oxidative damage ( Figure 4 ). The MDA, TG and LDL-C content was significantly higher in the DM groups than the control group (P<0.05), but significantly lower in the DM+β-carotene group (P<0.05). The SOD, GSH-Px, TC and HDL-C content was significantly lower in the DM groups than the control group (P<0.05), but significantly higher in the DM+β-carotene group than the control group (P<0.05).

Effects of β-carotene on abnormal glucose and lipid metabolism, including total triglycerides (TG), cholesterol (TC), HDL-cholesterol (HDL-C) and LDL-cholesterol (LDL-C) levels.
MDA content in serum (A) and cardiac tissue (D). SOD activity in serum (B) and cardiac tissue (E). GSH-px activity in serum (A) and cardiac tissue (D). Serum TG (G), TC (H), HDL-C (I) and LDL-C (J) were measured throughout the study, and average levels were calculated. *P<0.05 vs. control group; #P<0.05 vs. 4-week DM group; @P<0.05 vs. 8-week DM group (n=10).
3.6 Cardiac-function recovery after β-carotene feeding
EF, FS and LVEDV were lower in the DM groups than the control group, whereas both LVPWs and LVPWd were higher at 4 weeks (P<0.05; Figure 5 ). EF and FS were higher at 4 and 8 weeks in the DM+β-carotene group than the diabetes groups (P<0.05). No significant correlation in LVESV was observed between the 4-week diabetes and the DM+β-carotene groups. In the 8-week groups, LVPWd was higher than that in the control group, whereas LVPWs was lower than that in the DM groups (P<0.05).

Cardiac dysfunction in diabetic rats.
(A) Representative M-mode images of diabetic rats and controls. Serial changes in (B) ejection fraction (EF), (C) fractional shortening (FS), (D) LV end-systolic volume (LVESV), (E) LV end-diastolic volume (LVEDV), (F) LV posterior wall end diastole (LVPWd) and (G) LV posterior wall end systole (LVPWs), assessed by echocardiography. *P<0.05 vs. control group; #P<0.05 vs. 4-week DM group; @P<0.05 vs.8-week DM group (n=10).
3.7 Changes in cardiac muscle microvascular parameters
At the end of the experiments, rats were administered insulin clamping with or without additional β-carotene for another 120 min after fasting for more than 8 h. Insulin infusion significantly increased cardiac MBV but did not affect cardiac MFV in the control group ( Figure 6A–C ). The β-carotene infusion caused a slight but significant increase in basal MBF without affecting the MFV. The MBV was slightly but not significantly lower during β-carotene infusion in the 0-min DM groups than the 4-week and 8-week groups.
3.8 Effects of β-carotene on mitochondrial respiratory synthase and mitochondrial respiratory function in T2DM rats
The Clark oxygen electrode method was used to detect the respiratory function of myocardial mitochondria, including three-state respiration, four-state respiration, the respiratory control rate, phosphorus-oxygen ratio (ADP/O); in addition, the electron transport chain complex I, II, III and IV enzymatic activity was determined [18]. The β-carotene treatment significantly improved the activity of myocardial mitochondrial electron transport complexes I, III and IV in diabetic rats ( Table 4 ). The mitochondrial respiratory chain is highly important for removing H+ from the mitochondrial matrix at or near the inner mitochondrial membrane. A decrease in state 3 respiration in cardiac mitochondria in diabetic rats was observed, whereas state 4 respiration appeared normal in the DM+β-carotene groups ( Figure 7A, B ). ADP/O and the respiratory control rate index both decreased in the DM group, but these effects were restrained in the DM+β-carotene group, thus indicating that the respiratory chain was repaired by β-carotene ( Figure 7C, D ).
Activity of the cardiac mitochondrial electron transport chain complex (ETC)
Activity (nmol/min/mg mt prot) | Complex I | Complex II | Complex III | Complex IV |
---|---|---|---|---|
Control | 4.81±0.71 | 0.61±0.21 | 1.97±0.26 | 2.57±0.41 |
4-week DM | 2.76±0.31* | 0.52±0.19 | 1.14±0.17* | 2.37±0.40* |
4-week DM+β-carotene | 3.46±0.46# | 0.52±0.17 | 0.75±0.22# | 1.73±0.28# |
8-week DM | 1.85±0.44* | 0.53±0.17 | 0.69±0.15* | 2.26±0.32* |
8-week DM+β-carotene | 3.91±0.61# | 0.56±0.19 | 1.31±0.21#∇ | 2.01±0.29#∇ |
Notes: *P<0.05 vs. control group; #P<0.05 vs. 4-week DM; ∇P<0.05 vs. 8-week DM. Data are shown as mean±SEM (n=10).

Effects of high glucose and β-carotene treatment on cardiac mitochondrial respiratory-chain parameters.
States 3 (A) and 4 (B) of respiration, RCI (C) and ADP/O index (D). Data are the mean±SEM for four animals from each condition studied. *P<0.05 vs. control group; #P<0.05 vs. 4-week DM group; ΔP<0.05 vs.8-week DM group (n=10).
3.9 Effects of different concentrations of β-carotene on primary cardiomyocyte viability with or without PA
The cytotoxicity of β-carotene in primary cardiomyocytes was evaluated with MTT assays after 24-h incubation. The concentration for 50% of maximal effect (EC50) of β-carotene in primary cardiomyocytes at 24 h was 57.91±4.90 μM ( Figure 8B ). Treatment with 50 μM β-carotene resulted in no significant cytotoxicity from 0 to 24 h in CCK-8 assays ( Figure 8C ), thus indicating that the tested concentration of β-carotene was not cytotoxic to primary cardiomyocytes.

Bioactivity of β-carotene in primary cardiomyocytes.
(A) Chemical structure of β-carotene. (B) Cardiomyocyte metabolic activity, analyzed with MTT assays after treatment with a β-carotene dose range (EC50, 57.91±4.9 μM; n=4). (C) Quantification of primary cardiomyocyte viability after treatment with β-carotene for different times. (D) Quantification of primary cardiomyocyte viability after treatment with increasing concentrations of PA. (E) PA decreases 2-NBDG uptake of cardiomyocytes with or without insulin (500 nM). (F) Quantification of cardiomyocyte viability after treatment with different doses of β-carotene with or without PA co-incubation. Data are presented as the mean±SEM. *P<0.05 vs. control group; #P<0.05 vs. IR group (n=10).
Next, we evaluated the effect of PA on primary cardiomyocyte viability with MTT assays. Glucose uptake was assessed to identify the optimal concentration for inducing the IR injury model. Cell proliferation was attenuated by PA in a concentration-dependent manner (P<0.05; Figure 8D ). Insulin (500 nM) treatment enhanced the 2-NBDG uptake in primary cardiomyocytes beyond that in the control groups (P<0.05; Figure 8E ). PA evoked IR without clear induction of cytotoxicity in a range from 150 to 350 μM. Therefore, we established IR model by exposing cardiomyocytes to 350 μm PA and 500 μm insulin was used as a positive control.
Glucose uptake assays were performed to quantified whether β-carotene inhibited IR-induced injury in cardiomyocytes. A significant decrease in 2-NBDG uptake of cardiomyocytes was observed with PA treatment, whereas β-carotene (10–50 μM) and metformin (5 μg/mL, positive control) both increased 2-NBDG uptake (P<0.05; Figure 8F ). Our results suggested that β-carotene improved the insulin sensitivity of PA-induced cardiomyocytes.
3.10 Effect of β-carotene on the insulin signaling pathway
To confirm that β-carotene improved PA-induced IR, we examined the levels of insulin-pathway-associated proteins with ELISA kits in primary cardiomyocytes. High glucose with or without PA treatment significantly decreased the level of GLUT4 and phosphorylated Akt (Ser473), and increased the level of phosphorylated IRS-1 (Ser307; Figure 9A ). Moreover, β-carotene administration significantly attenuated PA-induced changes in Akt and IRS-1 phosphorylation levels (P<0.05).

Effects of β-carotene on the insulin signaling pathway in insulin-resistant cardiomyocytes induced by PA after 24-h treatment.
(A) ELISA showing the p-AKT/AKT ratio in cardiomyocytes. (B) ELISA showing the GLUT4 level in cardiomyocytes. (C) ELISA showing the IRS-1 level in cardiomyocytes.*P<0.05 represents significant differences. Data represent the three separate experiments. Each ELISA value is an average of two measurements.
3.11 β-carotene improves mitochondrial biogenesis in PA-induced cardiomyocytes
To further investigate the effect of β-carotene on cardiomyocyte mitochondrial function, we conducted PCR-based assays allowing for quantitative assessment of mitochondria, on the basis of the mitochondrial DNA (mtDNA) copy number. The ratio of mtDNA to nuclear DNA was significantly lower in PA-treated cardiomyocytes than in the control group ( Figure 10 ). Thus, mitochondrial crista depletion and the mtDNA copy number decrease might have been associated with partial respiratory inhibition resulting from IR insult. In addition, β-carotene significantly increased the mtDNA copy number (P<0.05). PGC1-1β, NRF-1 and TFAM gene expression was determined by RT-PCR. Markedly higher PGC-1β and NRF1 mRNA and significantly lower TFAM mRNA was observed in the control group than the high glucose alone and PA cultured groups. Comparison of the results in the high-glucose and PA group indicated a clear increase in PGC-1β and NRF1 mRNA with β-carotene treatment (P<0.05). These data suggested a novel pathway in which β-carotene is associated with increased mitochondrial biogenesis in insulin-resistant cardiomyocytes.
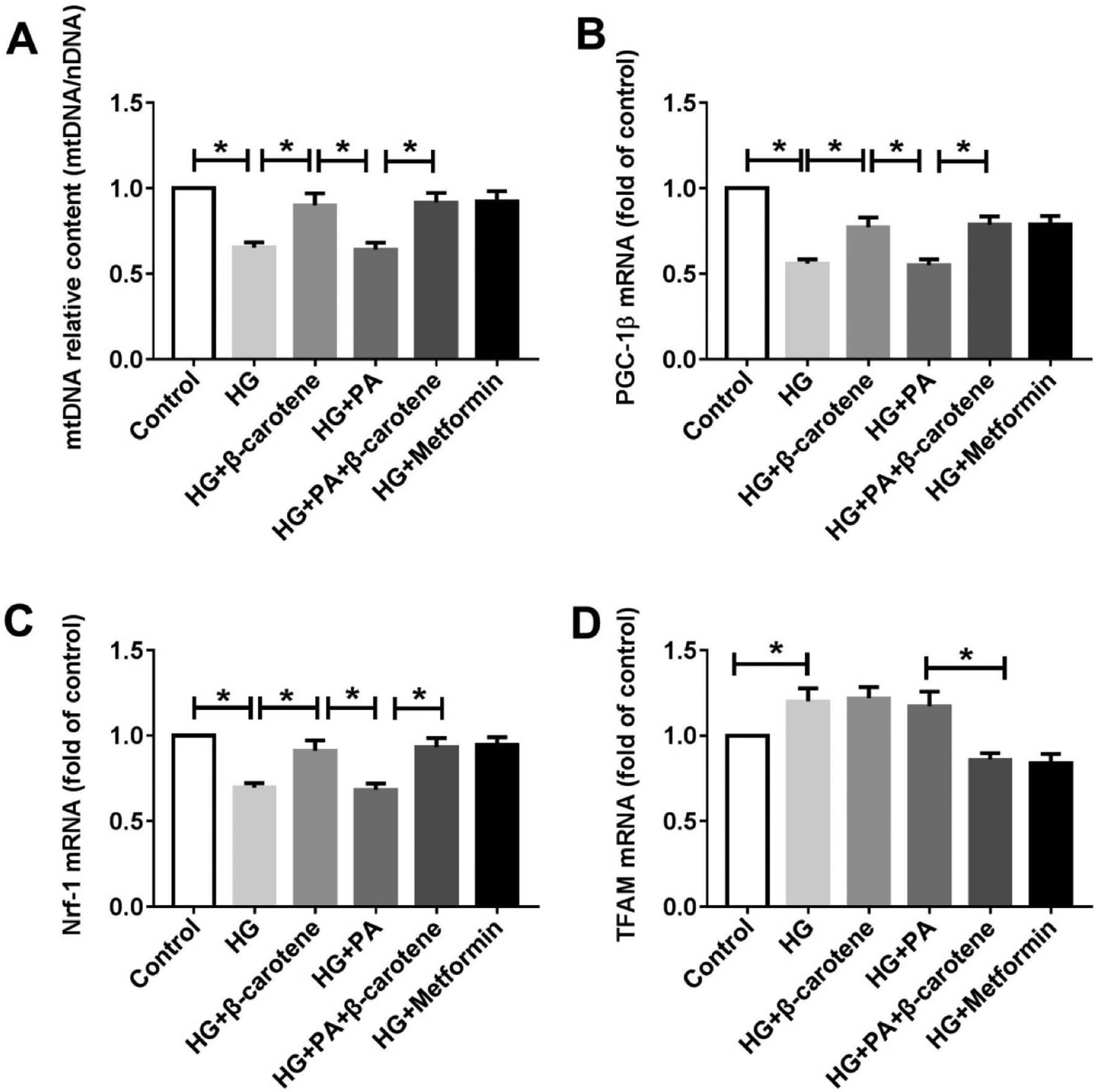
Effects of β-carotene on mitochondrial biogenesis in insulin-resistant cardiomyocytes induced by PA after 24-h treatment.
(A) Effect of β-carotene on the mtDNA copy number, determined with PCR. (B–D) Relative mRNA levels of PGC-1β, Nrf-1 and TFAM, determined by quantitative real-time PCR and calculated as the mean value with the comparative Ct method. Cardiomyocytes were treated with 5.5 mM glucose (lane 1, control), 25 mM glucose (lane 2, HG), HG+50 μM β-carotene (lane 3, HG+β-carotene), HG+350 μM PA (lane 4, HG+PA) and HG+350 μM of PA+50 μM β-carotene (lane 4, HG+PA+β-carotene) for 24 h. β-actin served as the loading control. *P<0.05 represents significant differences. Data represent the three separate experiments.
4. DISCUSSION
In recent decades, T2DM has increased in prevalence and become a major health concern worldwide [19]. Previous studies have presented limited evidence of the association between circulating vitamin C or carotenoids and the risk of T2DM, mainly because of the small numbers of participants examined [20, 21]. Our findings illustrated that: (i) serum cis-β-carotene levels are negatively associated with BMI, blood glucose and fasting insulin; (ii) β-carotene results in recovery of PA-induced cardiomyocyte mitochondrial biogenesis via an IR-related pathway; and (iii) β-carotene protects against diabetes through restoring glucose metabolism functions.
Carotene and structurally related compounds are precursors of vitamin A, which play important roles in the immune response, photosynthesis and cell differentiation. Therefore, carotenoids are widely used as dietary supplements with the aim of maintaining health and preventing diseases, such as cardiovascular disease, inflammation, diminished physical performance and mortality. However, large clinical trials have not demonstrated that tested formulas are beneficial, and some researchers believe that taking high-dose supplements may be harmful [22]. Vitamins A, D, K and E are considered relatively safe. Whereas physiological levels of these vitamins can be life-saving, megadoses can be dangerous [23]. High doses of vitamin D supplementation do not decrease the risk of vitamin deficiency; moreover, higher doses of vitamin E may increase cardiovascular disease and all-cause mortality [24, 25]. Research on carotenoids and their applications in DM is increasingly being performed, but the findings have been inconsistent. Little is known regarding the association between IR and serum carotenoids.
We used the NHANES 2001–2006 data, in which a total of 9281 participants were interviewed, to explore the relationship between β-carotenoids and glucose metabolism. In Table 1 , participants with the highest quartile (Q4) of cis-β-carotenoid levels had lower BMI (P<0.01), blood glucose (P=0.046) and fasting insulin (P<0.01) than those in the lowest quartile (Q1). Our findings emphasized that high circulating levels of cis-β-carotenoid reduced blood glucose level, increased blood insulin and improved insulin resistance. In addition, higher cis-β-carotene (Q4) levels were associated with higher LDL-C but lower TG levels ( Table 1 ). Pearson analysis also demonstrated a negative relationship between cis-β-carotene and TG ( Table 2 ). However, T2DM rat experiments showed inconsistent results with those in Table 1 . T2DM rats with β-carotene treatment showed diminished total TG and LDL-C levels, possibly because of the relatively small number of animals used. Furthermore, our findings did not appear to fully mimic the features in humans, and these analyses did not take several patients taking statins or other medicines into account.
Insulin resistance begins before the onset of T2DM in vivo and is the best predictor of T2DM onset. Previous studies have shown that strategies to decrease IR limit the development of T2DM and particularly ameliorate diabetic cardiomyopathy. Drugs such as resveratrol [26], trehalose [27], curcumin [28] and liraglutide [29] have been shown to promote autophagy in IR cardiomyocytes and diabetic animal models in various ways, thereby decreasing cell death. Epidemiological studies have indicated that diabetes is an independent risk factor that induces cardiac dysfunction, which is characterized by cardiac hypertrophy and diastolic dysfunction. We established a T2DM model to demonstrate that β-carotene treatment restored cardiac function and mitochondrial biogenesis in cardiomyocytes in vivo and in vitro ( Figures 5–7 ). IR and vascular dysfunction are strongly associated with the development of cardiovascular complications, which are the major causes of mortality in patients with T2DM. Peroxide and other ROS produced in vascular cells may play important roles in the pathogenesis of common vascular diseases [30, 31], particularly diabetes. Long-term clinical observational studies on diabetes have revealed that, in addition to metabolic toxicity factors, protective factors may also protect the function and survival of cells involved in microvascular diseases through influencing blood glucose control [32–34]. Therefore, we also examined cardiac microvascular recruitment on the basis of the diastolic/systolic stress MBF ratio, as a novel diagnostic index with moderate diagnostic accuracy [35]. Similarly to previous studies, our study demonstrated that adding β-carotene increased MBF and significantly increased mitochondrial respiratory function ( Figure 7 ).
Some anti-diabetic drugs can sufficiently decrease blood glucose levels but do not improve cardiac prognosis in patients with T2DM [36]. This finding may be due, at least in part, to a lack of understanding of cardiac IR. IR has been implicated in the pathogenesis of diabetic cardiomyopathy [37], even if it occurs only locally in the heart. Myocardial IR not only interferes with the metabolism of cardiomyocytes but also leads to mitochondrial dysfunction and oxidative stress in cardiomyocytes. Mitochondrial function is further regulated by the transcription factors PGC-1α, NRF1 and TFAM, which regulate key mitochondrial enzymes that affect mtDNA synthesis [38]. Long-term exposure of cardiomyocytes to high glucose increased the concentrations of superoxide ( Figure 4A–F ) and simultaneously increased mitochondrial damage ( Table 4 and Figure 7 ). Our results indicated that β-carotene can be used to treat glucose metabolic disorders, through its activation of PA-induced mitochondria biogenesis in diabetic rats and cardiomyocytes ( Figure 10 ).
5. LIMITATIONS
First, our data were obtained from a cross-sectional sample from 2001–2006 NHANES data and used to explore the relationship of β-carotene with glucose and lipid metabolism. We speculated that factors might affect blood glucose, insulin and HOMA-R. The immune responses or protein levels in humans should be interpreted with appropriate caution in prospective studies.
Second, because of limited statistical power, some associations might have been missed, such as behavioral habits and lifestyle factors, ethnic differences, smoking, and alcohol and coffee consumption, which were not adjusted for.
Third, the sample size was decreased because of missing, incorrect or incomplete blood glucose and cis-β-carotene data in the NHANES database.
Finally, owing to genetic and socioeconomic differences between the US and other countries, the generalizability of our findings might have been affected by the study’s exclusion criteria. Further longitudinal studies including additional factors that could potentially influence myocardial protection in terms of the association between β-carotene and glucose/lipid metabolism are required to provide replication.