1. INTRODUCTION
According to the World Health Organization, cancer is the second leading cause of death globally and is responsible for the deaths of approximately 10 million people annually [1]. Moreover, cancer morbidity is likely to increase substantially in the coming decades [2]. Despite substantial efforts to improve anti-cancer treatments, the situation remains grim. Metastasis and tumor resistance to current clinical drugs are two of the greatest obstacles [3, 4]. Therefore, developing new therapeutic approaches is crucial.
Ferroptosis is a unique type of programmed cell death that is morphologically, biochemically, and genetically distinct from other well-known forms of cell death [5]. It can be triggered by cellular redox homeostasis, and the resulting accumulation of iron-dependent lipid peroxidation products is the primary cause of cell death. Lipid peroxidation causes the plasma membrane to rupture, and cancer cells in epithelial-to-mesenchymal transition are particularly susceptible. This peroxidation promotes the destruction of metastatic tumors and persistent cancer cells, thus providing new therapeutic options for tumors that are resistant to existing therapies [6–8]. Moreover, owing to their vigorous metabolism and proliferation, cancer cells have a greater iron demand than normal cells and consequently are more susceptible to ferroptosis [9]. Therefore, ferroptosis selectively kills persistent cancer cells and has shown promise in decreasing the multiple drug resistance and metastasis of cancer cells.
Photodynamic therapy (PDT) is a promising anti-tumor therapy that uses reactive oxygen species (ROS) produced by photosensitizers (PSs) to kill cancer cells [10]. In comparison to conventional approaches, PDT has substantial advantages of on-demand administration, non-invasiveness, and avoidance of drug resistance. Importantly, it is a local treatment strategy causing minimal damage to healthy tissue [11–13]. In addition, PDT boosts antitumor immunity by enhancing intra-tumoral T lymphocyte infiltration and secretion of numerous pro-inflammatory factors, such as IFN-γ, thus contributing to the long-term inhibition of cancer cell proliferation [14]. Therefore, PDT is substantially superior to traditional treatments, such as surgery and chemotherapy, which are not only immune unresponsive but even immunosuppressive [15, 16].
Nevertheless, ferroptosis and PDT each have limitations. For example, the extremely short half-life of 1O2 and the hypoxic intratumoral microenvironment continually limit the efficacy of PDT [14, 17, 18]. Because high levels of inducers are required to trigger ferroptosis, the toxicity to normal tissues would be severe. However, these two strategies cooperatively elicit synergetic antitumor effects. Cellular ferroptosis enhances the efficacy of PDT by generating ROS through Fenton or Fenton-like reactions (Fe2+ + H2O2 → Fe3+ + (OH)− + •OH) that continuously produce oxygen [19]. In addition, PDT also drives ferroptosis by triggering non-enzymatic lipid peroxidation with generated ROS [18, 20]. Ferroptosis and PDT have synergistic rather than additive effects. Therefore, the combination of ferroptosis and PDT has high research value in anti-tumor therapy, particularly in resolving multidrug resistance and tumor metastasis [21].
In recent years, the combination of these two cancer treatment strategies has attracted extensive attention and shown broad application prospects for tumor treatment, particularly in solid tumors resistant to traditional therapies [22]. This review summarizes the research frontiers and development trends in this field, and systematically compares and discusses the strengths and limitations of these studies suggesting novel strategies for cancer therapy ( Scheme 1 ).
2. FERROPTOSIS COMBINED WITH PDT TO REGULATE INTRACELLULAR REDOX HOMEOSTASIS
ROS are by-products of aerobic respiration under physiological conditions. ROS cause DNA damage and genomic instability, thus driving the accumulation of tumor genetic alterations and promoting cancer progression [23]. Cancer cells require considerable ROS to support their invasive development [24]. However, massive ROS cause cancer cell death through DNA damage and through lipid peroxidation, commonly known as oxidative stress [25]. To cope with these conditions, the antioxidative system works to maintain cellular redox homeostasis. Glutathione (GSH), an essential component of the intracellular antioxidant system, participates in numerous metabolic activities and physiological processes in the human body. As an antioxidant, GSH scavenges excess ROS by being oxidized to GSSG either directly or via an enzymatic reaction [26]. To adapt to cell growth and survive under oxidative stress, tumor tissue contains four times more GSH than normal tissue [27].
In addition to regulating oxidative stress, GSH plays an important role in drug detoxification, thus posing hurdles for chemotherapy or PDT in anticancer therapy. GSH mediates the detoxification of cisplatin, doxorubicin, and other agents, thus resulting in anticancer resistance [28]. Although photosensitizers are excited to increase intracellular ROS, in cancer, intracellular GSH has direct quenching effects, thus impeding effectiveness [29]. Consequently, cancer therapies targeting GSH metabolism have been extensively studied.
GSH is synthesized in the cytoplasm from cysteine, glutamate, and glycine through two ATP-dependent enzymatic reactions [30]. Multiple pathways are involved in GSH synthesis and metabolism, as described in detail previously [30–33]. Here, we focus on the system xc --GSH-GPX4 pathway, which is also relevant to ferroptosis ( Figure 1 ). System xc - is a crucial glutamate/cystine transporter for cystine absorption. Cystine is the oxidized form of cysteine and a rate-limiting substrate for GSH synthesis. GSH-dependent lipid hydroperoxide glutathione peroxidase 4 (GPX4) converts lipid hydroperoxides to nontoxic lipid alcohols, thus hindering PDT and ferroptosis [34]. The amount of intracellular GSH determines the normal function of GPX4, and GSH deficiency also leads to the inactivation of GPX4 [35].
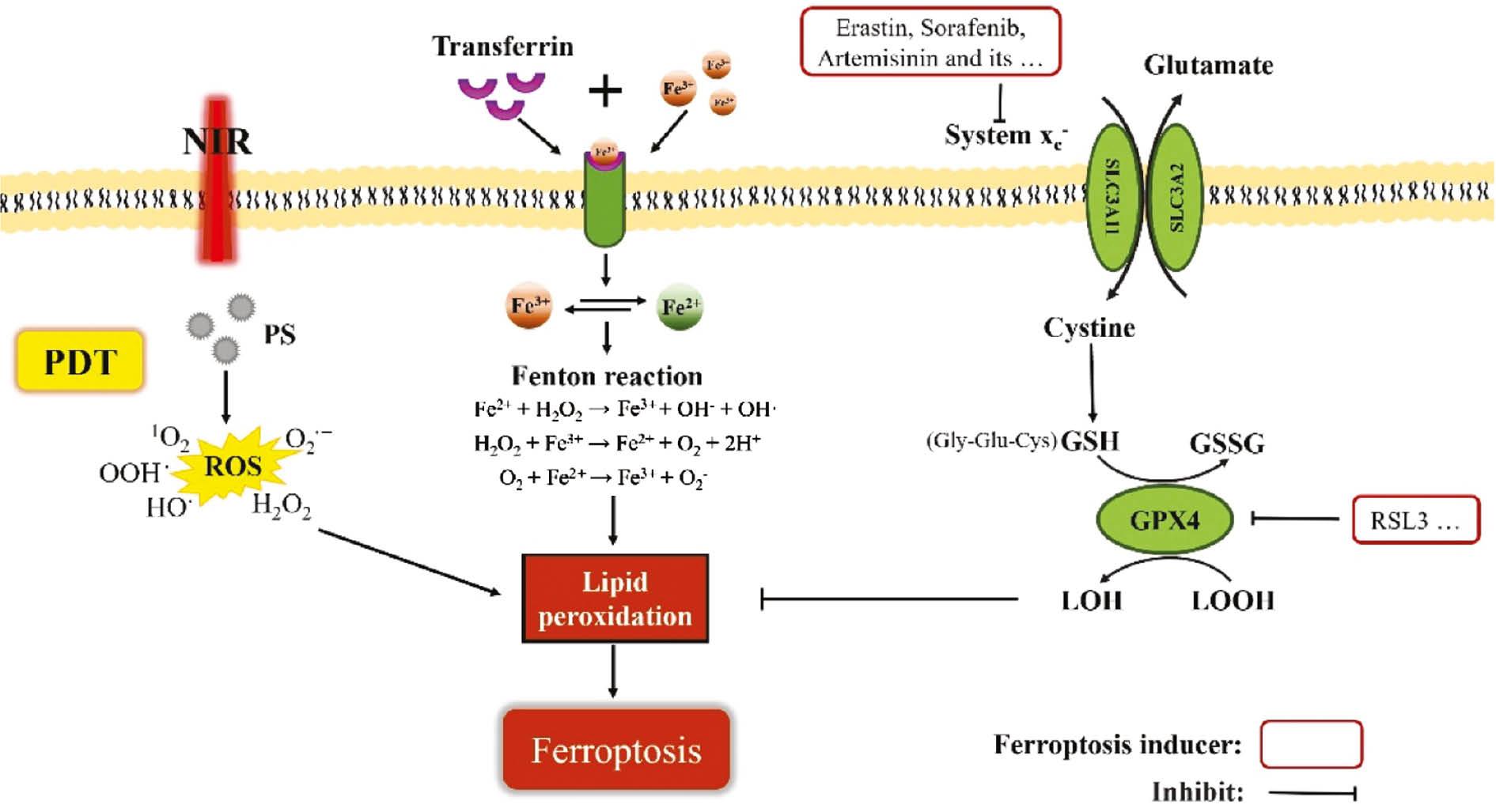
Schematic overview of ferroptosis combined with PDT to regulate intracellular redox homeostasis.
Intracellular GSH depletion increases cancer cell susceptibility to oxidative stress and chemotherapeutic drugs, and has also been shown to increase the efficacy of ROS-based therapy [31]. Ferroptosis inducers disrupt the GSH-redox system by blocking system xc - or inhibiting GPX4 [36]. In addition, PDT generates substantial amounts of ROS, thereby disrupting the intracellular redox balance. Therefore, PDT and ferroptosis cooperatively regulate the intracellular redox balance by affecting the metabolism of ROS and GSH, thereby enhancing each other’s anticancer efficacy. In the next section, we categorize these strategies according to the various ferroptosis triggering methods: mono-PDT and PDT-induced ferroptosis, combining PDT with small-molecule ferroptosis inducers, and combining PDT with metal-ion-induced ferroptosis ( Table 1 ).
3. CURRENT STRATEGIES FOR COMBINING FERROPTOSIS WITH PDT
3.1 Mono-PDT and PDT-induced ferroptosis
PDT is a revolutionary anticancer strategy that eliminates tumor cells by light-exciting photosensitizers to generate massive amounts of ROS. In contrast, the ROS produced by PDT initiate peroxidation of membrane lipids, thus leading to cellular ferroptosis, which is also characterized by uncontrolled iron-catalyzed lipid peroxidation [36, 37]. Hence, to some extent, mono-PDT can have synergistic effects in conjunction with the intrinsic iron pool [20]. Consequently, single-PDT-induced ferroptosis has been extensively studied, and ferroptosis inhibitors have been used to confirm the involvement of ferroptosis in tumor suppression beyond the effects of ROS generated by PDT [38–40].
Zhang et al. have used sinoporphyrin sodium to study cholangiocarcinoma and confirmed that PDT alone downregulates the expression of the cystine/glutamate transporter (system xc -), thus inducing ferroptosis [41]. In addition, to enhance the therapeutic effect, Wang et al. have used a polylactic acid-glycolic acid copolymer to load the photosensitizer IR780 ( Figure 2 ) [42]. The micelle nanoparticles were then coated with human osteosarcoma cell membranes to obtain a biomimetic active targeting nanoplatform (MH-PLGA-IR780). The nanoplatform promotes ferroptosis by inactivating GPX4, and its effectiveness has been demonstrated in human osteosarcoma-bearing mice. Similarly, Guo et al. have synthesized a Ce6 derivative (PS-NO) containing an NO donor [43]. High levels of NO produce reactive nitrogen species, which consume GSH and then disrupt intracellular redox homeostasis, thus causing oxidative stress and increasing cellular ROS levels [44]. Consequently, cancer progression is impeded through cell ferroptosis, and tumors are rendered vulnerable to chemotherapy, radiation, or immunotherapy [45]. The enhanced antitumor efficacy of PS-NO on the basis of the synergistic effects of ROS and reactive nitrogen species has been verified in Hela cells. Zhong et al. have loaded curcumin (as a photosensitizer) on gold nanorods (Au NRs) [46], then encapsulated Au NRs with upconversion nanoparticles (UCNPs) and modified them with phenylboronic double ester (PBE). Under near-infrared laser irradiation, the Au NRs/Cur/UCNPs@PBE elicit efficient photothermal therapy (PTT) and PDT. Further exploration of the mechanism mediating cell death has revealed that excess intracellular 1O2 depletes GSH and causes ferroptosis beyond apoptosis. This study has indicated the possibility of integrating PDT, PTT, and ferroptosis in a single nanoplatform with high performance.
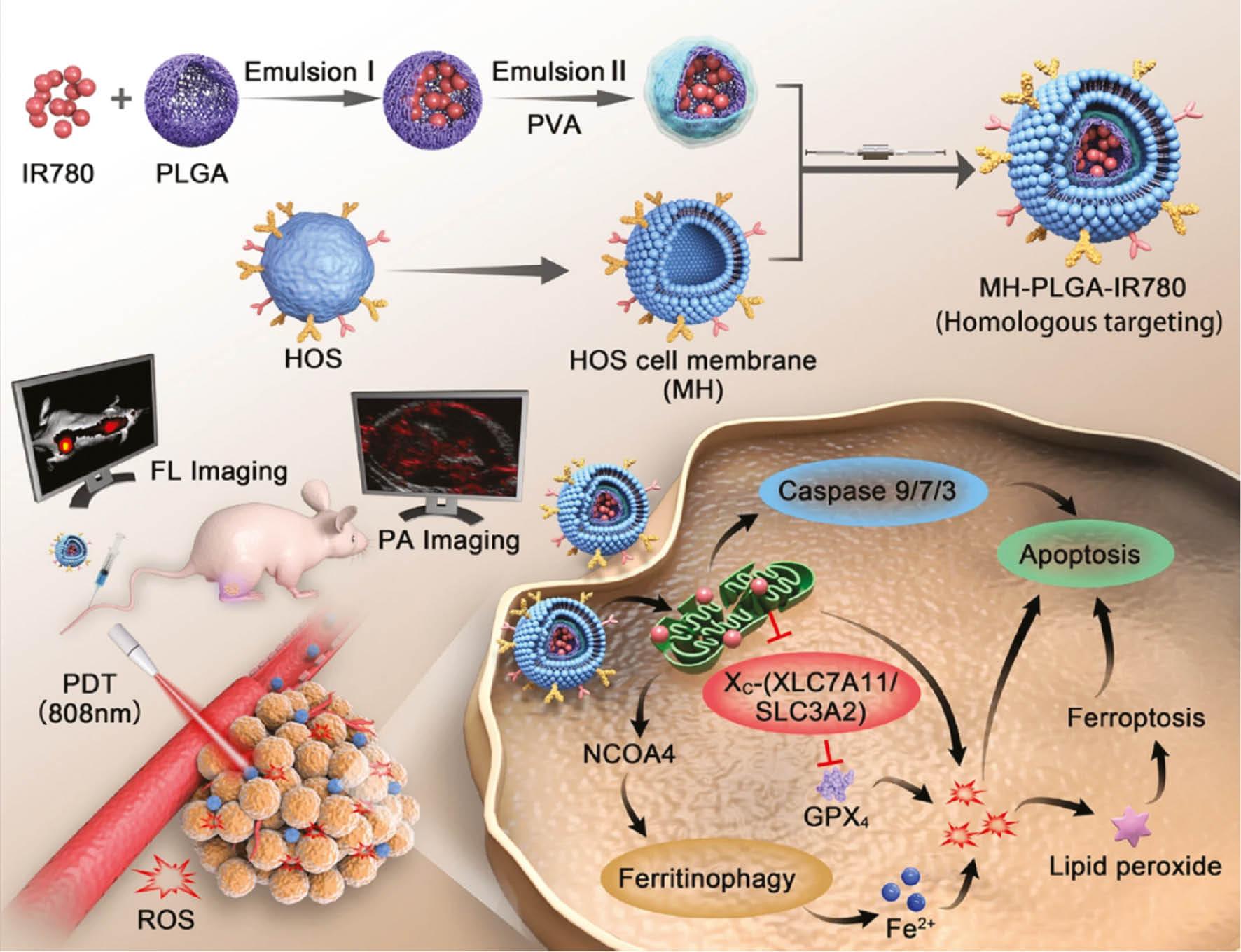
Schematic diagram of the fabrication and anticancer mechanism of MH-PLGA-IR780 (abbreviations: HOS: human osteosarcoma cell, FL: fluorescence, PA: photoacoustic). Reproduced with permission, [42] copyright 2022 Springer Nature.
GSH deficiency may inhibit GPX4 activity and further improve the efficacy of ROS-based therapies [31, 45]. However, mono-PDT cannot always produce optimal therapeutic effects, possibly because the PDT-induced DNA damage response upregulates GPX4 and further degrades PDT-induced ROS [47]. Furthermore, PDT-induced ferroptosis may be weak. In addition, the tumor’s hypoxic microenvironment would also hinder the efficacy of PDT. Combining photosensitizers with ferroptosis inducers selectively directs the development of cancer cells toward ferroptosis, thereby improving the anticancer effect of PDT [36].
3.2 Combining PDT with small-molecule ferroptosis inducers
As stated previously, the system xc --GSH-GPX4 pathway substantially affects the biosynthesis and metabolism of GSH as well as the cell anti-oxidation system ( Figure 1 ). Small-molecule ferroptosis inducers often act on this pathway and disrupt the cellular antioxidant system, thus causing accumulation of lipid peroxides. These ferroptosis inducers exacerbate oxidative stress in PDT by increasing ROS and abrogating antioxidation. In addition, PDT-induced ROS directly trigger lipid oxidation [31, 36, 37, 48].
3.2.1 Sorafenib
Sorafenib (SRF) is an approved antitumor drug by the U.S. Food and Drug Administration. SRF directly inhibits system xc -, thus resulting in GSH depletion and GPX4 inactivation. When SRF coexists with iron-rich sources, the ferroptotic effect is amplified several times [49]. Several studies have examined the synergic cancer treatments using SRF and photosensitizers with different carriers. For example, Xu et al. have incorporated Ce6 with hemoglobin (Hb) to construct a 2-in-1 nanoplatform (SRF@Hb-Ce6) loaded with SRF ( Figure 3a ) [49]. As an endogenous protein, Hb carries oxygen and iron. The Hb-based nanoplatform provides iron for iron-dependent ferroptosis and oxygen for oxygen-dependent PDT. The encapsulation efficiency of SRF can reach 70%. To obtain tumor-specific drug release, an amphiphilic matrix metalloproteinase 2 (MMP-2)-responsive peptide has been inserted into a Hb-Ce6 micelle skeleton. HIF-1 expression is significantly diminished at tumor sites after Hb-Ce6 treatment ( Figure 3b–c ), thus indicating its hypoxia-allaying effect. The intracellular singlet oxygen level is enhanced with Hb-Ce6 group compared with Ce6 alone, owing to the oxygen-supplying effect of the Hb-based carrier. In addition, the synergistic mechanism of SRF-induced ferroptosis and Ce6-induced PDT has been extensively investigated, and significant interference with the intracellular redox balance has been observed. GSH levels in the laser group (SRF@Hb-Ce6 (L)) were approximately 50% lower than those in the PBS group but were only 30% lower without laser treatment ( Figure 3d ). Lipid ROS (malondialdehyde) with SRF@Hb-Ce6 (L) were approximately 2-fold higher than those with SRF@Hb-Ce6 ( Figure 3e ). These results have verified the interaction between PDT and ferroptosis produced by the nanoplatform. Beyond the influence on intracellular redox balance, a synergistic effect has also been observed in the tumor immune response. SRF@Hb-Ce6 infiltrates the tumor tissue by recruiting many T lymphocytes, thereby activating the immune system and leading to secretion of IFN-γ. Subsequently, IFN-γ decreases SLC7A11/SLC3A2 expression, significantly decreases tumor volume, and increases mouse survival rate ( Figure 3f ). In vivo results have indicated a significant decrease in tumor volume and a significant increase in overall survival ( Figure 3g–i ). Similarly, Liu et al. have designed a nanoplatform (Ce6@SRF@RDV) for the co-delivery of Ce6 and SRF to cancer sites by using a red-blood-cell-derived vehicle (RDV) [50]. RDV has multiple functions similar to those of Hb. Furthermore, the membrane structure of the RDV enables cellular uptake and biocompatibility. This novel nanosystem has higher cellular uptake rates, enhanced PDT and ferroptosis effect as well as improved safety compared with that without membrane coated. In another study, Wang et al. have constructed a novel hypoxia-responsive nanoreactor (BCFe@SRF) [51] by combining Ce6 with bovine serum albumin and ferritin via an azobenzene linker conjugate, then loading SRF. BCFe@SRF is degraded by PDT in a hypoxic environment, thus releasing ferritin for catalysis of the Fenton reaction, and destroying tumor antioxidant defenses by SRF, thereby enhancing antitumor effects.
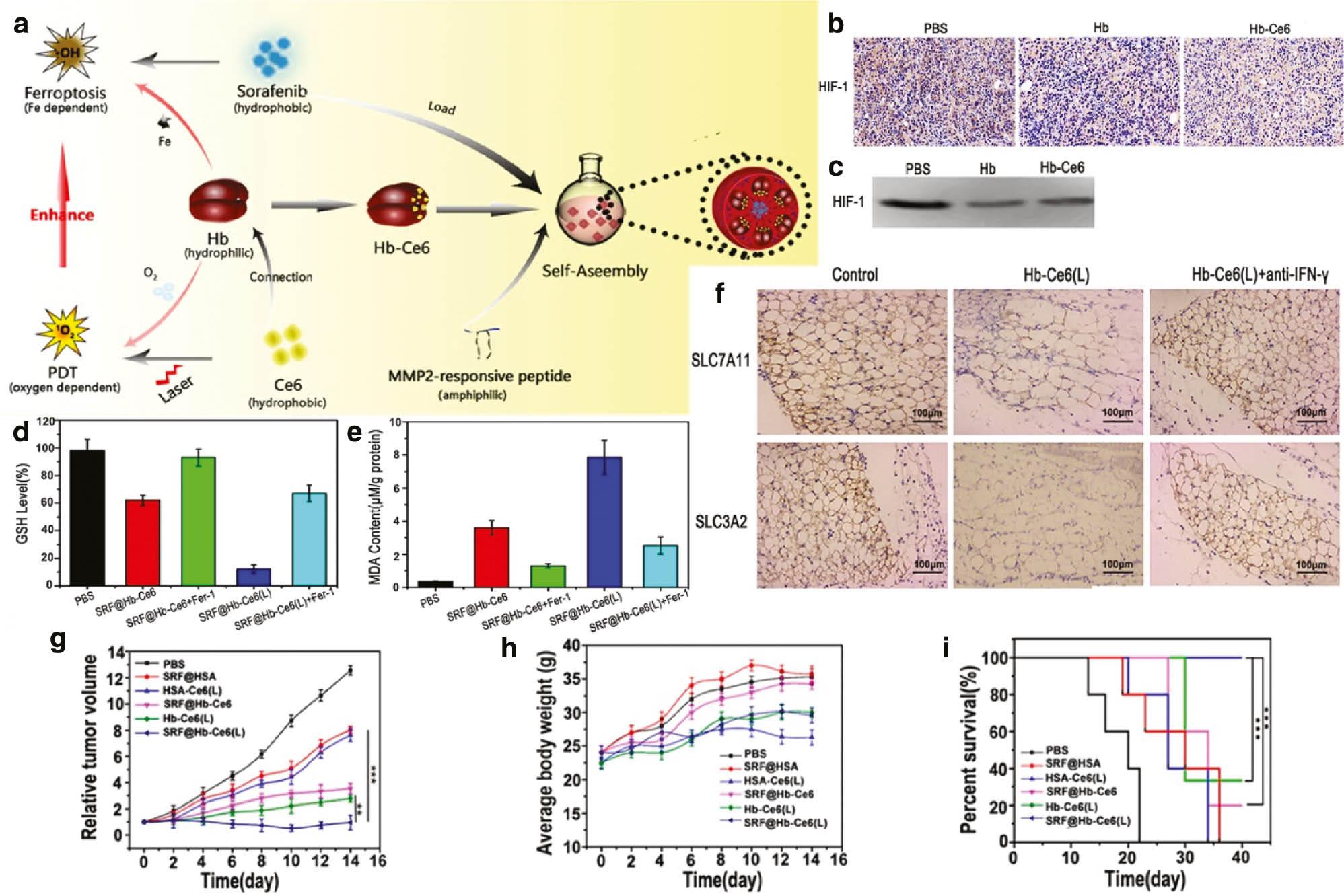
(a) Schematic diagram of the fabrication and anticancer mechanism of SRF@Hb-Ce6. (b) Immunohistochemical assay of HIF-1 expression in tumor tissues in the PBS, Hb, and Hb-Ce6 treatment groups. (c) WB assay of HIF-1 expression at the cell level in the PBS, Hb, and Hb-Ce6 treatment groups. (d) GSH level in tumor tissue, determined with DTNB assays. (e) MDA content in tumor tissue. (f) Immunohistochemical assays indicating the expression of SLC7A11 and SLC3A2. (g) Tumor growth curves of mice during the 14 d treatments. (g) Animal weight changes in different groups during the treatment period. (i) Survival rates of mice in different treatment groups. Reproduced with permission, [49] copyright 2020 American Chemical Society.
3.2.2 RSL3
RSL3 is a ferroptosis activator that induces ferroptosis in tumor cells by downregulating GPX4 [52]. RSL3 and PDT have been reported to induce immunogenic cell death (ICD) [16]. Song and colleagues have developed acidity-activatable dynamic nanoparticles that boost ferroptosis and PDT, and further promote cancer immunotherapy [16]. The nanoparticle (BNP@R) was fabricated by integration of a pyropheophorbide A (PPa) containing copolymer and an acid-labile copolymer for tumor-specific delivery of RSL3 ( Figure 4a ). BNP@R is activated by the tumor acidic microenvironment and triggers anti-tumor immune responses via PDT and GPX4-associated ferroptosis. The authors treated tumor cells with their developed BNP@R, then incubated them with IFN-γ, and observed greater ferroptotic cell death than that elicited by BNP@R alone, thus suggesting that IFN-γ-induced GSH bleaching promotes lipid peroxidation ( Figure 4b–c ). In vitro results have also confirmed that BNP@R induces PDT-based ICD, and the lipid peroxidation generated by both the PDT and ferroptosis cumulatively promotes this ICD effect ( Figure 4d ). In vivo, the ability of BNP@R to promote intra-tumoral infiltration of CD8+ T lymphocytes has also been validated ( Figure 4e ). In addition, the nanoparticles developed in combination with PD-L1 blockade further enhance tumor immune response and inhibit metastasis ( Figure 4f–h ).
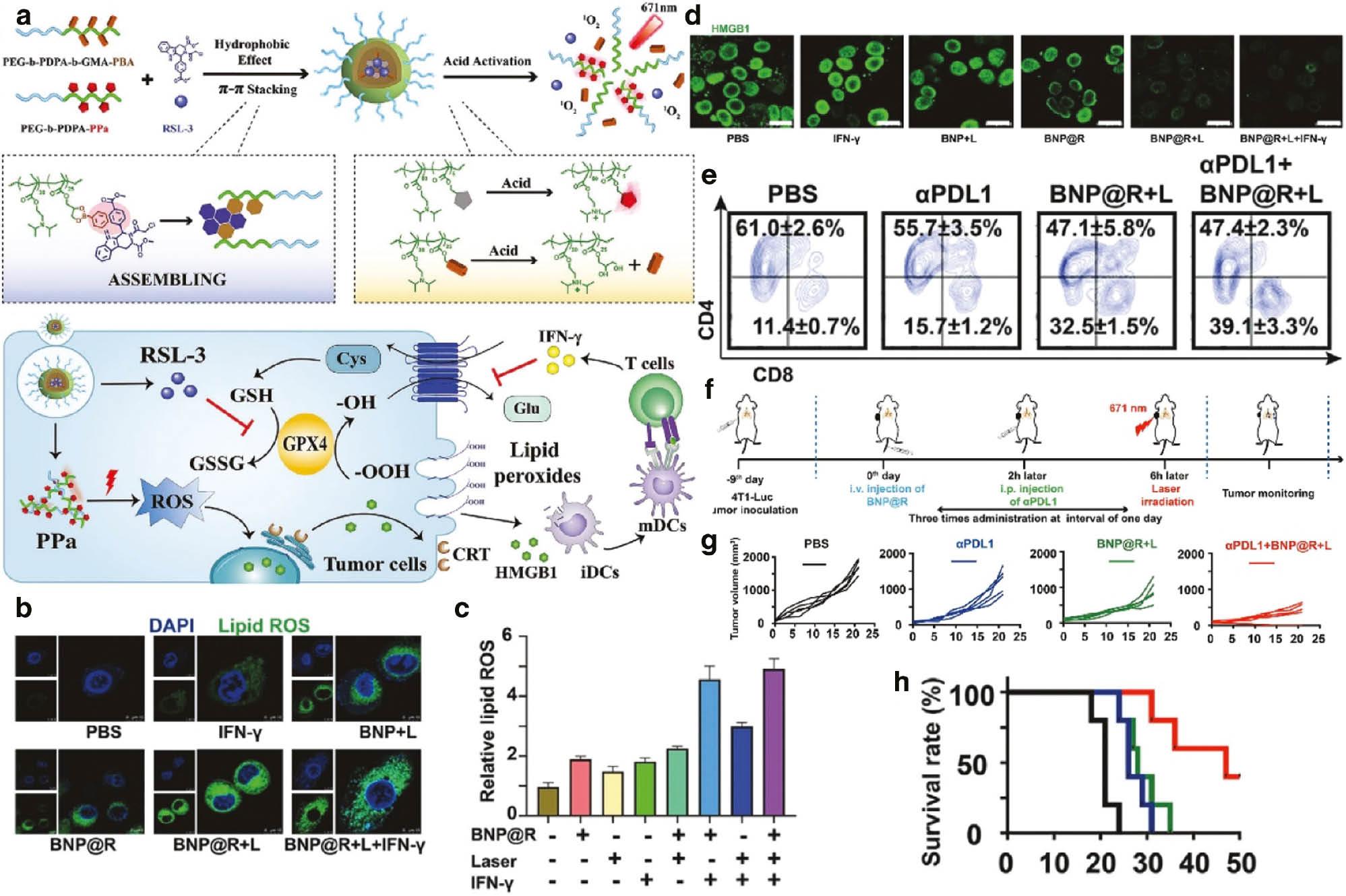
(a) Schematic diagram of the fabrication and anticancer mechanism of BNP@R. (b) CLSM examination and (c) flow cytometric analysis of PDT and GPX4-inhibition-induced intracellular accumulation of lipid peroxides in B16-F10 tumor cells in vitro with a BODIPY-C11 fluorescent probe. (d) Flow cytometric examination of ferroptosis-induced CRT expression on the surface of the of B16-F10 cell membrane in vitro. (e) Tumor-infiltrating CD8+ and CD4+ T lymphocytes in total CD3+ T cells. (f) Treatment schedule for PD-L1 blockade and nanoparticle-mediated ferroptosis induction in mice bearing 4T1 breast tumors. (g) Tumor growth and (h) survival curves of 4T1-tumor-bearing BALB/c mice subjected to different treatments. Reproduced with permission, [16] copyright 2021 John Wiley and Sons.
Although the combination of ferroptosis and PDT-induced immunotherapy holds promise for long-term tumor control, several issues remain to be resolved. The inflammatory response of phagocytes may inhibit antitumor immunity and stimulate tumor growth [53]. Thus, further research is required to elucidate the distinct roles of both in tumor immunity. Few known PSs induce potent ICD, except for hypericin, 5-aminolevulinic acid, Rose Bengal (RB) acetate, and glycoconjugated chlorin; therefore, new PSs that induce ICD must be developed. To this end, Turubanova and colleagues have synthesized two cyanol porphyrazines resembling porphyrins and phthalocyanines; these tetrapyrrole macrocycles have both high photodynamic activity and strong immunogenicity [54]. Future studies may use these new PSs combined with ferroptosis to elicit enhanced tumor immune responses.
3.2.3 Erastin
In 2012, erastin was found to induce cancer cells to undergo ferroptosis, a unique form of non-apoptotic cell death [55]. Numerous studies have since demonstrated that erastin induces GSH depletion by inhibiting system xc - function [48, 56–58]. Zhu and colleagues have developed a self-assembling nanoplatform composed of erastin and Ce6 [19]. In comparison to single Ce6-based PDT, Ce6-erastin nanoparticles increase tumor cell ROS. Ce6-erastin nanoparticles also significantly enhance antitumor effects. In another example, Du et al. have loaded erastin and a PS (RB) into exosomes (Er/RB@ExosCD47) to deliver both compounds to tumor sites with high specificity [59]. Exosomes have shown effective tumor distribution via CD47, thereby eliciting a “do not eat me” signal. In addition, the promising in vivo antitumor effect of Er/RB@ExosCD47 have been confirmed, and combining ferroptosis inducers with PSs has been verified to enable use of a lower drug dose, thereby decreasing adverse effects.
3.2.4 Dihydroartemisinin
Beyond SRF, many drugs on the market have been demonstrated to induce ferroptosis. Dihydroartemisinin (DHA), a sesquiterpene lactone compound derived from the natural plant Artemisia annua, downregulates the expression of GPX4 and triggers ferroptosis in lung cancer cells [60]. Han et al. have found that the oxidative stress caused by Ce6 upregulates GPX4, thus degrading ROS and leading to drug resistance [47]. However, the downregulation of GPX4 by DHA-induced ferroptosis significantly enhances PDT efficacy. In vitro results have also shown that a combination of DHA solution and Ce6 solution has good anti-lung cancer efficacy, decreasing cell viability by 3 times and increasing the apoptosis rate by 3 times, thus providing a solid theoretical foundation for the improvement of PDT anti-lung cancer efficacy with the traditional Chinese medicine DHA.
3.2.5 Buthionine sulfoximine
Glutamate-cysteine ligase (GCL) is an enzyme that mediates GSH synthesis [31]. Buthionine sulfoximine (BSO) is a GCL inhibitor that decreases GSH levels by blocking GSH synthesis. Yoo et al. have combined Ce6-loaded nanoparticles (Ce6-PEG-PLA-NPs) with BSO [61]. Laser exposure to BSO more than doubles the accumulation of Ce6 in tumor tissue at each point in time, while decreasing GSH concentrations. This treatment significantly decreases the viability of cancer cells, as shown in SCC-7 cells. In vivo results have indicated that the combination of the preparation and BSO completely inhibits tumor growth within 14 days without causing substantial changes in mouse body weight.
In general, combining small-molecule ferroptosis inducers with PDT shows promise in improving redox interference-based cancer treatments. Among the various combination strategies, we expect that RDV or exosomes mainly based on biomimetics such as Hb may achieve better therapeutic effects because of their biocompatibility and internal functions. Furthermore, strong immune responses evoked by PDT aided by ferroptosis in these studies would shed new light on multimodal therapies (PDT-ferroptosis-immunotherapy) for achieving satisfactory therapeutic effects in cancer therapy.
3.3 Combining PDT and metal-ion-induced ferroptosis
Iron ions are indispensable in many physiological processes in the human body, and are relatively safe with low toxicity. Ferroptosis is an iron-dependent cell death [62] in which iron plays two main roles: (1) Sufficient iron constitutes an active pool, which produces large amounts of hydroxyl radicals through the Fenton reaction. The hydroxyl radicals promote the formation of lipid peroxides in a non-enzymatic manner [63]; (2) The lipoxygenase family members are the main enzymes that mediate lipase oxidation, and these lipoxygenases depend on iron supply to maintain their activity [64]. Therefore, interventions affecting iron homeostasis in tumor cells and the iron-overload state are also strategies to induce ferroptosis [9]. Hence, several studies have focused on the combination of iron-ion-based ferroptosis and PDT.
A tannic acid (TA) network with a polyphenol structure has been shown to reduce Fe3+ to Fe2+ [65, 66]. Exploiting this property, Yu et al. have designed a self-assembling nanoplatform (p53/Ce6@ZF-T, Figure 5a ) [67]. The nanoparticles are composed of a p53 plasmid-complexed Ce6-poly(amidoamine), Fe2+-containing mesoporous zeolitic imidazolate framework-8, and TA. After endocytosis by tumor cells, Fe2+ is released from the p53/Ce6@ZF-T and reacts with local H2O2, thereby generating toxic •OH via the Fenton reaction. TA, with its strong reductive ability, ensures the continuous supply of Fe2+ from Fe3+. By-products from the Fenton reaction, such as O2, alleviate hypoxia and therefore enhance Ce6-induced PDT ( Figure 5b ). Both the Fenton reaction and PDT amplify oxidative stress, thus leading to lipid peroxidation of the plasma membrane ( Figure 5c–d ). Moreover, the complexed p53 plasmid in p53/Ce6@ZF-T decreases SLC7A11 expression and suppresses GPX4 activity, thus promoting ferroptosis ( Figure 5e ). In vitro and in vivo studies have demonstrated remarkable antitumor efficacy.
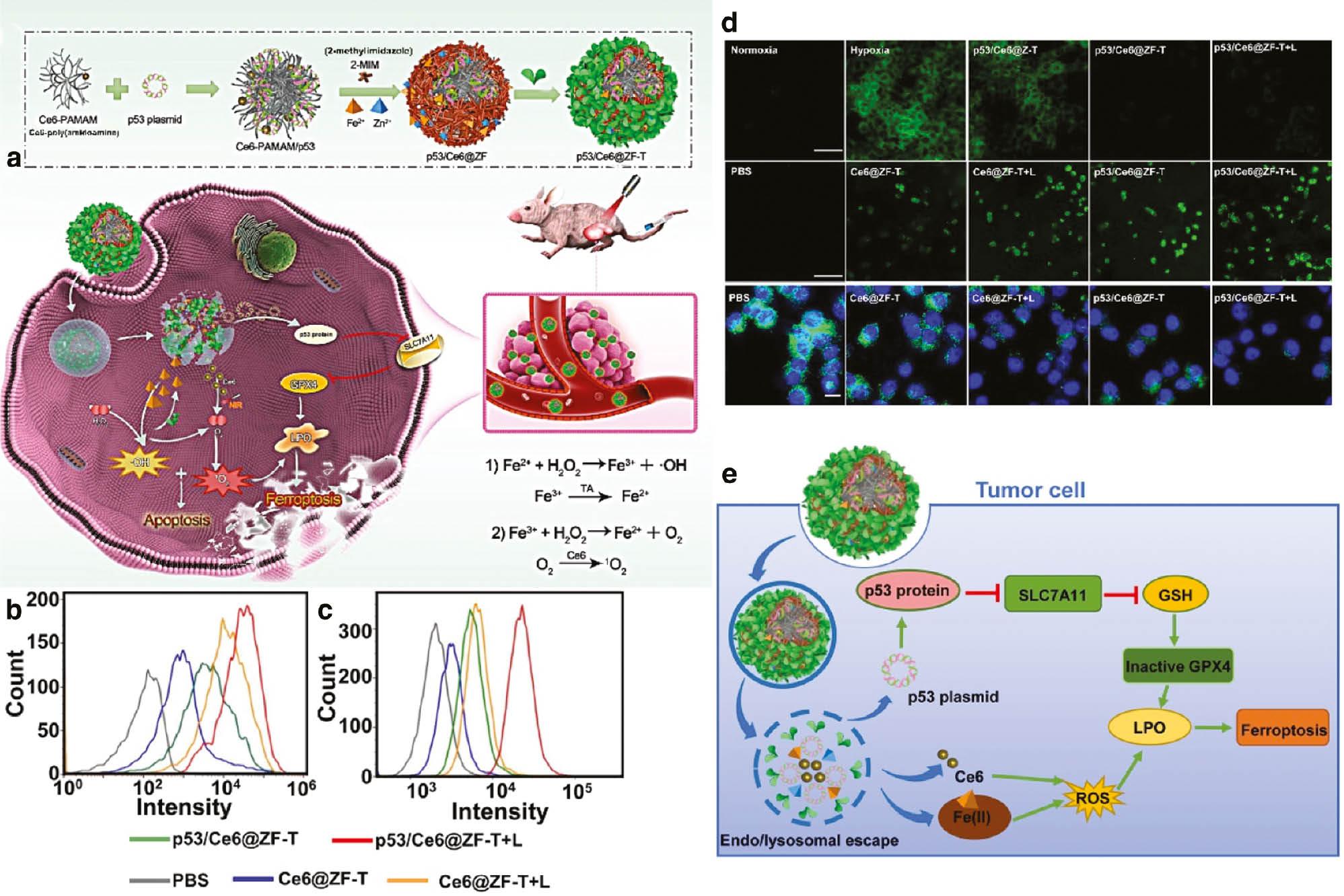
(a) Schematic diagram of the fabrication and anticancer mechanism of p53/Ce6@ZF-T. (b) Intracellular ROS levels, determined by flow cytometry in H1299 cells with different treatments. (c) Intracellular LPO generation, determined by flow cytometry. (d) CLSM images of H1299 cells after incubation under normoxia with PBS or hypoxia with different treatments. (e) Illustration of the mechanism of p53/Ce6@ZF-T induced ferroptosis. Reproduced with permission, [67] copyright 2022 Elsevier.
The donors of iron are mostly inorganic particles, which may not be biocompatible and may lead to safety concerns. Chen et al. have directly combined hemin and Ce6 into nanoparticles (HCNPs) through self-assembly [68]. Hemin, the prosthetic group of the Hb, is composed of proto-porphyrin and ferrous atoms. Hemin has multiple advantages, such as Fenton catalytic activity and GSH-depletion capability [69–71]. HCNPs have been validated to generate both O2 and •OH, thus enhancing the PDT effect. In addition, HCNPs show strong GSH depletion ability, thus triggering Fe2+-dependent ferroptosis. HCNPs have been found to have greater antitumor efficacy than Ce6 alone, thereby indicating their efficiency.
Some researchers have taken a different approach of using synthesis or preparation methods to directly endow iron with photodynamic properties. Yang et al. have developed a simple, rapid, and inexpensive synthetic method to make ferric ions into hydrated iron oxide (ferrihydrite) [72]. Ferrihydrite releases large amounts of Fe2+ under blue light irradiation, which promotes irreversible DNA fragmentation and ROS-associated ferroptosis. Similarly, Cheng et al. have used Au and Ag to fabricate Fe3+-loaded nanoclusters (Fe3+@Au1Ag24@PbP) [73]. Under near-infrared laser irradiation, Fe3+@Au1Ag24@PbP not only achieves the combined therapeutic effects of PDT and ferroptosis, but also increases the temperature of the tumor microenvironment and generates a photoacoustic signal.
As previously described, ferroptosis is a regulated cell death associated with lipid oxidation. Studies by Perez’s group have shown that addition of polyunsaturated fatty acids induces ferroptosis in human cancer cells [74]. Given this, Liang et al. have engineered Fe3O4, porphyrins, and lipids into nanoparticles (Fe3O4@PGL NPs) [75]. They have found that extremely low doses of nanoparticles effectively destroy cancer cells, thus verifying the synergistic effects of the three substances.
The tumor microenvironment is hypoxic and mildly acidic with excess GSH and H2O2, thereby markedly affecting therapeutic effects [76–79]. Manganese oxide nanomaterials and their derivatives have unique properties, such as catalyzing H+/H2O2 to oxygen (O2), thereby relieving tumor hypoxia [80]. Chen et al. have used black phosphorus nanosheets (BNPs) to anchor MnO2 nanoparticles and Fe3O4 nanoparticles, then coated them with M1 macrophage membranes to form an intelligent biomimetic nanoplatform [81]. Fe3O4 nanoparticles induce ferroptosis, and BNPs mediate PDT under 670 nm laser irradiation, thus exhibiting synergistic antitumor effects. In addition, MnO2 in the nanoplatform serves as an oxygen-generating reactor that enhances PDT efficacy. This combination of treatments has elicited strong anticancer effects and prolonged survival in tumor-bearing mice, through overcoming the tumor barrier (hypoxia) and enhancing M1-macrophage membrane-mediated tumor-targeting capability.
In addition to the commonly used iron, manganese, platinum, and copper, additional metal-based materials, including tellurium, molybdenum, cobalt, gallium, indium, and cerium, have been reported to save PDT-based ROS by depleting GSH and inducing ferroptosis [31]. Using metallic osmium, Lu et al. have developed an osmium-peroxygen complex (Os2) that is inactive in the dark but releases the peroxyl radical O2•− under light, even in the absence of oxygen, and converts into a cytotoxic osmium complex (Os1) [82]. In hypoxic tumors, Os1 is cytotoxic with or without radiation. Meanwhile, light-activated Os2 induces photocatalytic oxidation of endogenous 1,4-dihydro-nicotinamide adenine dinucleotide in living cancer cells, thus leading to GSH degradation, GPX4 downregulation, and lipid peroxide accumulation, and triggering ferroptosis. Moreover, in vivo studies have confirmed that Os2 effectively inhibits the growth of hypoxic solid tumors in mice. Similarly, Ke et al. have proposed an easy oxidative polymerization technique for biodegradable iridium (IrIII) coordination polymers (IrS NPs) [83]. Under light induction, IrS NPs generate singlet oxygen and superoxide anion radicals, which selectively accumulate in mitochondria, thus causing mitochondrial dysfunction and fragmentation, and triggering cell death through mixed apoptotic and ferroptotic pathways. Thus, the resistance to traditional therapeutic drugs can be overcome. This therapy has the potential to eradicate tumors. Yuan et al. have also synthesized a benzothiophene isoquinoline-derived cyclometalated iridium complex (MitoIrL2) by using iridium [84]. MitoIrL2 produce large amounts of 1O2 after light irradiation, which in turn cooperate with the released iridium in inducing ferroptosis, thus providing a novel opportunity to overcome hypoxia-induced resistance in PDT.
Iron ions play key roles in inducing ferroptosis. Various types of strategies based on iron materials are widely used for ferroptosis, such as iron oxide, ferrihydrite iron-organic frameworks, and amorphous iron NPs. Unlike small-molecule ferroptosis inducers, these iron materials can be nano-sized or used as nano-carriers by themselves [85–87], thus providing advantages in combination with PDT. In addition, some iron or metal materials can serve as both ferroptosis promoters and PSs, and consequently have received increasing interest in combined anticancer therapy. However, much more research, including investigations of adverse effects and interindividual differences, will be needed before these iron/metal-based materials can be applied in clinical settings [88–91].
Synergistic formulations based on ferroptosis and PDT
Preparation | Ferroptosis inducer | Photosensitizer | Cancer cell lines/animal models | References |
---|---|---|---|---|
Drug solution | / | Sinoporphyrin sodium | Cholangiocarcinoma | [41] |
MH-PLGA-IR780 NPs | / | IR780 | Osteosarcoma | [42] |
PS-NO solution | / | Ce6 | Cervical cancer | [43] |
Au NRs/Cur/UCNPs@PBE NPs | / | Curcumin | Melanoma | [46] |
SRF@Hb-Ce6 NPs | Sorafenib | Ce6 | Mouse breast cancer, mouse liver cancer | [49] |
Ce6@SRF@RDV NPs | Sorafenib | Ce6 | Osteosarcoma | [50] |
BCFe@SRF NPs | Sorafenib | Ce6 | Mouse liver cancer | [51] |
BNP@R NPs | RSL3 | PPa | Mouse melanoma, mouse breast cancer | [16] |
Ce6-erastin NPs | Erastin | Ce6 | Oral tongue squamous cell carcinoma | [19] |
Er/RB@ExosCD47 NPs | Erastin | Rose Bengal | Hepatic cancer | [59] |
Drug solution | DHA | Ce6 | Lung cancer | [47] |
Ce6-PEG-PLA-NPs & BSO | BSO | Ce6 | Mouse squamous cell carcinoma | [61] |
p53/Ce6@ZF-T NPs | Fe2+/p53 | Ce6 | Lung cancer | [67] |
HCNPs | Hemin (Fe2+) | Ce6 | Mouse breast cancer | [68] |
PEG-Fns | Ferrihydrite | Ferrihydrite | Mouse breast cancer | [72] |
Fe3+@Au1Ag24@PbP nanoplatform | Fe3+ | Au1Ag24 nanoclusters | Mouse liver cancer | [73] |
Fe3O4@PGL NPs | Fe3O4 | porphyrins | Colon cancer | [75] |
Drug solution | Os2 | Os2 | Cervical cancer | [82] |
IrS NPs | Ir-SH | Ir-SH | Lung cancer | [83] |
Drug solution | MitoIrL2 | MitoIrL2 | Pancreatic cancer, breast cancer | [84] |
4. FERROPTOSIS AND PHOTODYNAMIC THERAPY COMBINED WITH OTHER ANTITUMOR THERAPY
4.1 Chemotherapy
On the basis of the tumor hypoxia microenvironment, delivery systems have been designed from different perspectives. One perspective is to provide oxygen to alleviate hypoxia. For instance, the SRF@Hb-Ce6 [49] and HCNPs [68] use hemoglobin and hemin, respectively, to provide oxygen for enhancing PDT, and iron for ferroptosis and improved biocompatibility. Another perspective exploits tumor hypoxia. Pan et al. have used PDT to aggravate the hypoxic tumor microenvironment, thus further activating the prodrug tirapazamine for chemotherapy [92]. They have constructed a nanoplatform incorporating the hypoxic-responsive prodrug tirapazamine (TPZ) into a polyvinyl pyrrolidone dispersed nanoscale metal-organic framework of Fe-TCPP (where TCPP is tetrakis (4-carboxyphenyl) porphyrin). The framework was then coated with cancer cell membranes to obtain PFTT@CM, a tumor-targeting agent ( Figure 6a ). PFTT@CM has synergetic PDT and ferroptotic effects, as detailed illustrated in the above examples, including enhanced PDT due to the oxygen-supplying and •OH-generating Fenton reaction, GSH depletion, and increased lipid peroxidation ( Figure 6b–d ). In addition, TPZ in the nanoplatform has chemotherapeutic effects. The drug loading efficiency of TPZ is 27.1 ± 7.4%. With the abundant ROS generated under laser excitation, tumor cells have been found to be more hypoxic with than without laser treatment, thus inducing TPZ activation. Additionally, TPZ causes nucleic acid damage that kills tumor cells. PFTT@CM with laser irradiation elicits greater nucleic acid damage, than TPZ alone and PFTT@CM without irradiation ( Figure 6e–i ). These results have indicated that PDT in combination with hypoxic-responsive TPZ yields superior anticancer effects. Tumor growth is significantly suppressed by PFTT@CM with laser with synergistic therapeutic strategies (ferroptosis, PDT, and chemotherapy). Beyond loading of chemotherapeutic agents into synergistic drug delivery systems, recent studies have demonstrated that certain classical chemotherapeutic drugs, such as cisplatin and doxorubicin, induce ferroptosis-like cell death [7]. For example, Fang et al. have found that DOX promotes free-iron release, thus leading to ferroptosis [93].
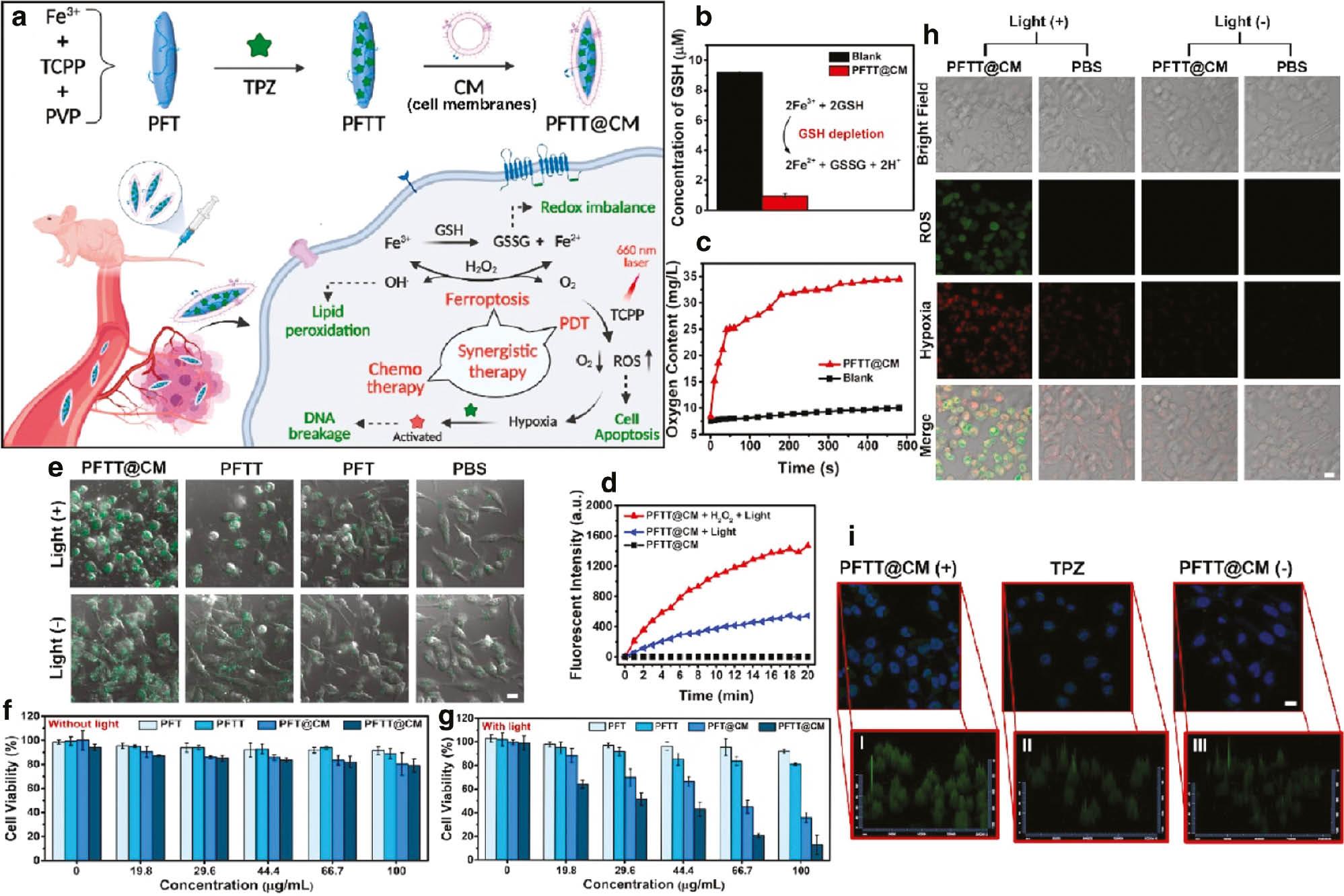
(a) Schematic diagram of the fabrication and anticancer mechanism of PFTT@CM. (b) GSH depletion ability of PFTT@CM, mediated by Fe3+. (c) Time-dependent oxygen generation ability of PFTT@CM in H2O2 solution. (d) SOSG fluorescence changes in the presence of PFTT@CM + H2O2 + light, PFTT@CM + light, or PFTT@CM only. (e) Apoptosis detection of MDA-MB-231 cells after various treatments, with Annexin V-FITC as an indicator. Dark (f) and light (g) toxicities of PFT, PFTT, PFT@CM, and PFTT@CM against MDA-MB-231, measured with CCK8 assays. (h) ROS and hypoxia conditions of MDA-MB-231 cells treated with PFTT@CM, with PBS as a control, under light irradiation and dark. (i) CLSM images of MDA-MB-231 cells pretreated with PFTT@CM with (+) or without (−) light and TPZ, followed by labeling with TUNEL reagents and DAPI. Reproduced with permission, [92] copyright 2022 Elsevier.
4.2 Photothermal therapy
PTT is a new anti-tumor therapy that uses photothermal agents to convert light energy to heat energy that kills tumor cells [94]. As a non-invasive treatment, PTT has the benefits of low toxicity, specificity, and repeatability [95]. PTT has substantial advantages over conventional tumor therapy [96]. Studies have shown that PTT enhances cellular uptake of Ce6, thus increasing the efficacy of PDT [97]. Therefore, PTT is frequently combined with PDT to enhance anti-tumor effects [98–100].
Tao et al. have constructed a self-synergistic nanoplatform for tumor therapy [101]. They have used a silica nano-network to integrate the nuclear factor erythroid 2-associated factor (Nrf2) inhibitor brusatol, manganese dioxide, and the PS Ce6 in the nanoparticles, which were coated with folate (PEG-FA)-modified polydopamine (PDA) (brusatol/silica@MnO2/Ce6@PDA-PEG-FA, BSMCPF). Ce6-mediated PDT induces high levels of ROS and stimulates Nrf2 expression, thus triggering antioxidant and antipyretic defense. Brusatol inhibits the activation of the Nrf2 pathway, thus inactivating GPX4 and ferritin heavy chain (FTH), enhancing PDT, and inducing ferroptosis. Compared with nanoparticles without brusatol, BSMCPF yields significantly higher levels of lipoxidation products and Fe2+. In addition, the PDA in the nanoparticles shows market PTT effects. BSMCPF has been found to increase the temperature in tumor tissues from 31 to 52 °C within 10 min. This nanoplatform exhibits promising antitumor effects with combinations of PDT, PTT and ferroptosis, and shows promise against hypoxia- and hyperthermia-associated resistance in anticancer treatments.
4.3 Sonodynamic therapy
The non-invasive treatment modality ultrasound (US)-triggered sonodynamic therapy (SDT) overcomes the barrier of the low tissue penetration depth of PDT [102]. Gan et al. have constructed a manganese porphyrin-based metal-organic framework (Mn-MOF), which triggers the release of oxygen (O2), enhances PDT, and decreases intracellular GSH, thereby inducing ferroptosis through US [103]. A study has confirmed that porphyrin-based MOFs can be designed for efficient PSs in PDT, owing to their to high PS loading capacity and an absence of self-quenching. Mn-MOFs inhibit tumor growth and metastasis. In addition, Mn-MOFs effectively reshape the tumor immune microenvironment by increasing the number of activated CD8+ T cells and mature DCs, and decreasing the numbers of myeloid-derived suppressor cells in tumor tissues.
Combining multiple therapeutic approaches into single agents to provide multi-modal delivery systems can decrease doses, avoid multidrug resistance, and achieve better efficacy [104]. However, optimizing the ratios of different drugs remains a challenge. The different metabolic rates of different types of drugs in different patients lead to complex pharmacodynamic/pharmacokinetic relationships, which require extensive clinical studies and leads to higher costs [105, 106].
5. CONCLUSION AND OUTLOOK
In conclusion, the combination of ferroptosis and PDT for cancer treatment has become a hotspots in the field of cancer therapy. Here, we summarized several strategies for ferroptosis combined with PDT, including mono-PDT and PDT-induced ferroptosis, combining PDT with small-molecule ferroptosis inducers, and combining PDT with metal-ion-induced ferroptosis. These examples demonstrate that PDT and ferroptosis have mutually reinforcing effects with high antitumor efficacy and safety, thereby providing a promising strategy for modern cancer treatment. Furthermore, we have presented other antitumor therapies in combination with these two treatments.
Studies have shown promising results in vitro and in vivo, but the design of drug delivery systems remains challenging [48]. First, the in-depth mechanisms of combined PDT and ferroptosis anticancer therapies must be further uncovered through various biochemical technologies, to provide a foundation for the future design of more effective and specific delivery systems. Second, although PDT is a controllable therapy with minimal invasiveness and adverse effects, ferroptosis may pose a risk of normal cell/tissue damage. Hence, developing combined delivery systems with tumor targeting ability for specific tumor microenvironments will be crucial. Third, significant differences exist between animal models and humans [107, 108], and further research on the correlations between them is required. Advances in technology such as organ-on-a-chip or organoids may be promising for evaluating these complicated synergistic strategies [109–111]. In addition, most studies have focused on the combined influence of PDT and ferroptosis on intracellular redox homeostasis. However, their ability to induce the tumor immune response makes these therapies attractive for treating cancer metastasis [112]; further studies are needed to provide detailed insights into their immunogenicity. Finally, because combination treatments contain several elements and must introduce different carriers, the safety problems of carriers cannot be overlooked. In addition to conventional methods, recent advances in microfluidic-assisted chemical synthesis and bioassays, as well as artificial-intelligence systems that improve design hypotheses through feedback analysis, will accelerate carrier screening and the development of preparations [104, 105, 113]. Therefore, further research will be needed to investigate synergistic therapies to develop formulations with superior efficacy and fewer toxic adverse effects.