BACKGROUND
The influenza A virus (IAV) has been detected in a wide range of host species, thus making it a prototype for emerging viruses with pandemic potential. Wild aquatic birds are the natural reservoirs of diverse influenza A viruses, and the corresponding infections are usually mild or asymptomatic. Avian influenza viruses (AIVs) can be transmitted to other species, such as domestic poultry, swine, and horses. Cross-species transmission of avian IAV to humans is rare. In the past decades, sporadic human infections with AIVs, including the H5N1, H5N6, H6N1, H7N2, H7N3, H7N4, H7N7, H7N9, H9N2, H10N7, H10N8, H5N8, and H10N3 subtypes, have been reported [1–3]. Many of these zoonoses are “dead end”: no subsequent transmission has been observed, and only a small number of cases have been reported. However, H7N9 IAV has been responsible for the major zoonosis in recent decades (Fig 1). The first laboratory-confirmed H7N9 human case was identified in eastern China in early spring, 2013 [4]. Recent exposure to poultry or visitation of live poultry markets (LPMs), where AIV can be maintained and amplified, are the main risk factors for human infection [5]. Fortunately, LPM closures effectively decreased the incidence of human infections [6]. This virus caused five continuous epidemic waves with a total of 1,568 cases and 616 deaths (case fatality rate of 39%). No human H7N9 cases have been reported after March 2019, probably because of the massive vaccination efforts in poultry after September 2017 in China [7]. The H7N9 influenza virus causes severe or fatal outcomes in humans but shows low pathogenicity in poultry, thus enabling “silent” evolution and transmission to humans. However, the emergence of the highly pathogenic H7N9 avian influenza (HPAI H7N9) in poultry, with increased virulence and an expanded host range to ducks, posed a new threat not only to poultry but also to public health [8,9]. Although no new human cases have been reported since 2019, the potential for sporadic human infections cannot be ignored, because H7N9 influenza viruses have continually been detected in poultry and related environments.

Human infections with avian influenza viruses in recent decades.
The cumulative number of cases of human infection with different avian influenza virus subtypes since the first reported year or the year of a well-known epidemic (H7N7). The size of the circle represents different cumulative numbers of cases: the smallest circle represents one reported case.
H7N9 IAVs undergo “genetic tuning” through reassortment, acquiring mutations in viral proteins that enable cross-species transmission from poultry to humans. However, human infections with the H7N9 virus remain rare, and limited human-to-human transmission has been reported; therefore, host genetic factors might also contribute to susceptibility to the H7N9 virus. Here, we summarize current findings regarding the viral and host factors that contribute to H7N9 IAV interspecies transmission.
ACQUIRED FEATURES OF VIRAL PROTEINS FOR ADAPTION TO MAMMALIAN HOSTS
H7N9 IAV is a triple-reassortant virus with two surface genes from different H7 and N9 viral subtypes, and all six internal genes from the H9N2 virus [4]. The early reassortment probably occurred in wild birds, and the second reassortment probably took place in domestic birds in east China in 2012, through exchange of internal genes from H9N2 viruses [10]. Those early strains underwent continual reassortment, and acquired amino acid substitutions in their viral proteins and features required for adaptation to mammalian hosts, such as greater binding affinity to “human-type” receptors. However, interspecies transmission from poultry to humans is not determined solely by the enhanced receptor binding ability in the cell-attachment process but is also associated with other altered viral-protein molecular markers, which might affect the transmission and replication of H7N9 influenza viruses.
H7N9 IAV enhanced “human-type” receptor binding properties
Host-cell attachment is the initial host-species barrier that can control or limit infection. The hemagglutinin (HA) protein, expressed on the surfaces of influenza viruses, binds terminal sialic acids (SAs), which are associated with the larger glycans on the surfaces of host cells, thus enabling viral internalization. In humans, α2,6-linked SAs are abundantly expressed in the upper respiratory tract (i.e. the nasopharynx), whereas a mixture of α2,3- and α2,6-linked SAs are present in the lower respiratory tract (i.e., the lungs and bronchi) [11,12]. Acquisition of a binding preference for α2,6-linked SA (the “human-type” receptor) in the HA protein is believed to be a fundamental feature underlying the adaptation of AIVs to mammalian hosts. As previously reported in various influenza viruses subtypes [13–15], the G186V and Q226L mutations (H3 numbering) in the HA receptor-binding site have been detected in H7N9 influenza viruses, and contribute to its enhanced “human-type” receptor binding preference [16]. Furthermore, substitutions of both G186V and Q226L, or G186V alone, have been detected in the HPAI H7N9 virus [17,18].
Neuraminidase is a sialidase protein that cleaves SA from infected cell surfaces, thus enabling the release of progeny virions for viral spreading [19]. The N9 of H7N9 viruses bears a short-stalk with a deletion of 5 amino acids at positions 69–73, which is commonly observed in terrestrial poultry [20]. Furthermore, N9 shows inefficient cleavage of α2,6-linked SA receptors, thus potentially compromising aerosol transmission in ferrets [21]. However, a recent study has indicated that N9 also has receptor binding properties mediated by a hemadsorption site in addition to an enzymatic site with enhanced binding to “human-type” receptors [22].
Therefore, the overall increase in α2,6-linked SA-receptor binding properties due to mutations in the HA protein as well as the additional neuraminidase-binding feature are possible advantages that may facilitate H7N9 influenza virus infection in human cells and mammalian hosts. Unlike H5N1 IAV, which has a binding preference for α2,3-linked SA (the “avian-type” receptor) and has only rarely cased human infections since 1997, H7N9 influenza virus has an enhanced α2,6-linked SA binding specificity, which might partially explain the larger number of human cases caused by this novel avian influenza virus [16]. H7N9 influenza viruses have acquired an ability to bind “human-type” receptors. However, they have retained their ability to bind α2,3-linked SA [23]. Furthermore, N9 shows poor cleavage activity toward α2,6-linked SA receptors, thus potentially limiting viral spread and release from human cell surfaces for further replication. These characteristics may explain why only limited human-to-human transmission has been reported [5,24,25] and why efficient airborne transmission of the H7N9 virus has not been observed in ferret models [26].
H7N9 IAV enhances replication or virulence in mammalian hosts
The genomic replication of the avian influenza virus is poor in mammalian cells. However, multiple molecular viral polymerase mutations that overcome this major species barrier have been reported. Polymerase basic protein 2 (PB2) is a major host determinant, and T271A, Q591K, E627K, and D701N substitutions have been reported in most LPAI and HPAI human H7N9 isolates [18,20]. Those mutations are associated with greater polymerase activity in mammalian cells or enhanced virulence in mice. The E627K or D701N mutations in PB2 are critical for the adaptation of AIVs to mammalian hosts [27–29]. These mutations increase the polymerase activity and viral replication of H7N9 viruses in vitro and in vivo [30–32]. Although no human isolates with dual mutations in PB2 at positions 627 and 701 have been reported, these mutations have been discovered in a ferret transmission model and have also been found to confer enhanced fitness in mammalian cells and mice [33]. Another subunit of the polymerase basic protein PB1 has a potentially biologically important I368V mutation [34,35], which is associated with acquired transmissibility of the H5N1 avian influenza virus among ferrets [36]. The PB1 gene also encodes the full-length PB1-F2 protein of approximately 89 amino acids. However, some H7N9 isolates encode only shorter-length PB1-F2 proteins, as also observed in other influenza A viruses [20]. This shorter length is associated with greater pathogenicity in mice, but its contribution to the virulence of H7N9 viruses remains unclear. Moreover, human-like signatures have been reported, including PA V100A, K356R, and S409N mutations [20].
HOST FACTORS CONTRIBUTING TO SUSCEPTIBILITY TO H7N9 INFECTION
Human genetic determinants of H7N9 avian influenza viral infections
Epidemiological investigations have revealed that exposure to poultry is the most likely independent risk factor for human H7N9 infections. In one study, 73% (887/1220) of infected patients were found to have been exposed to infected poultry and/or contaminated environments, mainly LPMs, whereas only 7% (91/1220) of the confirmed cases were associated with occupational exposure to poultry [37]. Furthermore, serological surveys of poultry workers have indicated undetectable or limited neutralizing antibodies against the H7N9 virus (seropositivity rate of 0.11–0.93%) [38,39]. Additionally, in the general population, two sero-epidemiologic surveys between 2013 and 2016 have reported zero or very low seropositivity rates for H7N9 influenza virus [38,40]. Because human infections with H7N9 viruses remain very rare, host genetic factors might also determine susceptibility to H7N9 infections.
Previous studies have shown that host genetic factors are important in the pathogenesis of, or susceptibility to, influenza viral infections [41]. Single-nucleotide variants (SNVs) of several genes, including TMPRSS2, IFITM3, TLR3, and CD55, are associated with the severity of human infections with H7N9 viruses [42–44] (Table 1). The rs4820294/rs2899292 haplotype GG of LGALS1 is associated with protection from H7N9 infections, possibly because of significantly higher LGALS1 mRNA and protein expression in lymphoblast cell lines [45]. However, little is known regarding genetic predisposition to H7N9 infection. In addition, most SNVs identified in single-variant analyses have high minor-allele frequencies (MAF > 5%, gnomAD) in humans. Furthermore, with the development of next-generation sequencing, rare SNVs predisposing humans to H7N9 infection can be identified through human whole-genome sequencing. For example, a low-frequency variant of UBXN11 and three HLA alleles (DQB1*06:01, DQA1*05:05, and C*12:02) have been associated with H7N9 infection in human [46]. Moreover, our recent investigation of the contribution of rare mutations in poultry workers frequently exposed to H7N9 viruses, compared with other populations, has identified 17 defective SNVs in the myxovirus resistance 1 (MX1) locus in H7N9-infected patients, without sex differences, on the basis of whole-genome sequencing [47]. In vitro experiments also demonstrated that 14 of the 17 MxA protein variants, including H7N9, H7N7, and H5N1, had lost the ability to inhibit avian IAV replication. These results demonstrate the key role of MX1-based antiviral defense in controlling interspecies transmission. Additionally, previous studies have shown that human MxA inhibits influenza virus replication by blocking the nuclear translocation of incoming viral ribonucleoproteins with the help of additional cofactors to achieve anti-influenza activity [48]. To verify the antiviral mechanism of human MxA and how missense mutations in different domains influence its antiviral effects, further studies are needed. Such studies would improve understanding of how AIVs cross species barriers.
Host genes associated with human susceptibility to H7N9 infections.
Host gene | Single-nucleotide variant (rsID) | Variant class | Animal studies | Reference(s) |
---|---|---|---|---|
IFITM 3 | rs12252 | Common variants(MAF >5%) | + | [43,44] |
TMPRSS2 | rs2070788/rs383510 | Common variants(MAF >5%) | + | [42] |
LGALS1 | rs4820294/rs2899292 haplotype GG | Common variants(MAF >5%) | + | [45] |
CD55 | rs2564978 | Common variants(MAF >5%) | − | [43] |
UBXN11 | rs189256251 | Low-frequency variants(0.5% < MAF <5%) | − | [46] |
MX1 | c.88C>T rs138644617 rs764277171 rs368357662 rs141166870 rs779947445 rs762946488 rs754725725 c.439C>A c.757G>A rs1172769444 rs370594768 c.1589T>G c.1625T>C | Rare variants(MAF <0.5%) | + | [47,55] |
MAF, minor-allele frequency in East Asian individuals in the Genome Aggregation Database v2.2.2 (gnomAD); (+) validated and (−) not validated in mouse models.
Male sex bias of H7N9 infection
Interestingly, men have consistently accounted for approximately 70% of all H7N9 infection cases in the past five epidemic waves [37]. Previously, differential healthcare-seeking/access behavior between men and women was proposed to contribute to sex biases in the case distributions in surveillance and diagnosis [49]. However, a modeling study has revealed that increased exposure time in LPMs does not increase the risk of H7N9 infections in older men, thus suggesting that underlying biological host factors between sexes may influence the susceptibility and severity differences in H7N9 infections [50]. Interestingly, a mouse model has indicated that female mice have greater weight loss and lower survival rates than male mice after H7N9 infection [51]. Although no sex-specific differences in viral titers have been observed in the lung, female mice show a greater inflammatory response than male mice. Similarly, observations regarding the currently circulating SARS-CoV-2 virus also indicate the importance of sex differences: male sex is a risk factor for hospitalization [52,53]. A recent study has also identified that perturbed sex-hormone metabolism might be a hallmark of critically ill men with Coronavirus disease 2019 [54]. In contrast to the pandemic of zoonotic SARS-CoV-2 infections, to which both sexes are susceptible, H7N9 infections show no or limited human-to-human transmission, but display a very apparent bias toward males. Hence, further investigation of the underlying mechanisms of sex-dependent disease outcomes of H7N9 infections is needed to guide patient-centered clinical management.
CONCLUSIONS
Studies have revealed mutations in viral proteins that overcome the host barrier and host factors that increase the susceptibility to H7N9 infections in humans (Fig 2). Although no H7N9 human cases have been reported, this virus is still occasionally detected in poultry or contaminated environments. Further viral adaptations, such as stronger human-receptor binding ability, may still pose a threat for a future pandemic, and individuals with susceptible host genes may develop severe outcomes after infection. Many scientific questions remain to be addressed. For example, what are the underlying mechanisms of male-biased H7N9 infections? What are the potential mechanisms of discovered host genes, such as MX1? Understanding the mechanisms of viral adaptive changes and host susceptibility might provide new potential targets for better, more precise preparation for future zoonoses leading to epidemics or pandemics.
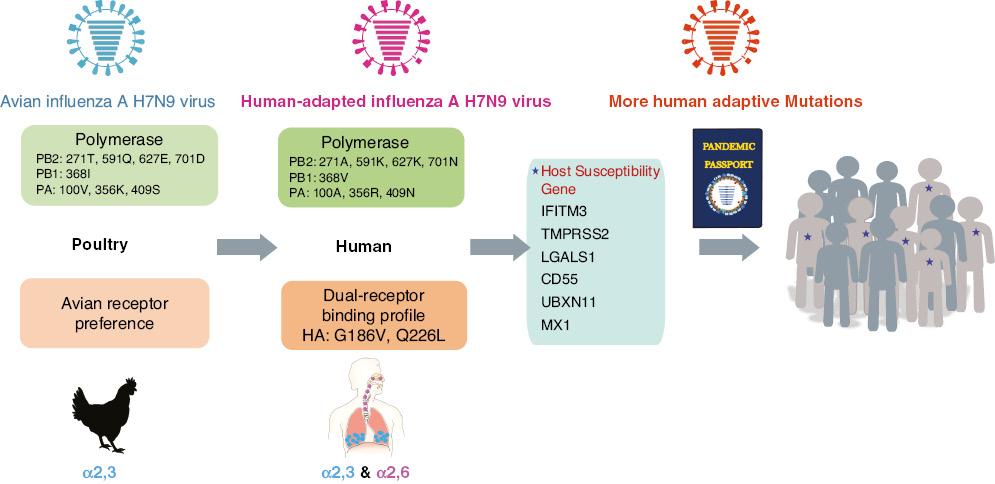
Viral and host factors overcome the cross-species barrier in human H7N9 infections.
Mutations in viral proteins and host factors shown to increase susceptibility to H7N9 infections in humans. Further adaptation of the H7N9 virus poses a continued pandemic threat. The presence of susceptible host genes may be associated with poorer disease outcomes.