Introduction
The emergence of multidrug-resistant bacteria is a global health threat [1, 2]. New antibacterial alternatives are urgently needed for combating drug-resistant bacteria [3]. Antimicrobial peptides (AMPs) are generally short and positively charged peptides that widely exist in various organisms (bacteria, fungi, animals, and plants) [4, 5], and are considered as the potential alternative of traditional antibiotics against bacteria owing to their broad-spectrum antimicrobial properties and low tendency to drug resistance [6]. AMPs are usually composed of cationic and hydrophobic amino acids in an amphiphilic structure and kill bacteria mainly through the disruption of microbial cell membrane [7, 8]. The cationic domains of AMPs attach to the anionic constituents of bacterial membrane through electrostatic interaction, and then the hydrophobic regions insert into bacterial lipid layer, leading to a disruption of cell membranes [9, 10].
Currently, several AMPs are in the market and clinical applications, such as gramicidin [11] and polymyxins [12]. However, most of them are intended for topical treatment but seldom for infections in deep tissues such as muscle layers or any part of organs [13]. This is mainly because of their undesirable toxicity and low bioavailability in vivo [14]. AMPs cause severe systemic toxicity due to their non-specific membranolytic activity toward normal tissue cells, which is the main obstacle in clinical development [15]. For instance, polymyxins can cause severe nephrotoxicity and neurotoxicity through intravenous administration [16]. AMPs are highly susceptible to proteolysis and can be rapidly cleared in vivo, which also restrict their application [14, 17].
Much effort has been put to improve the efficacy of AMPs while reducing their toxicity, including chemical modification [18] and the use of delivery vehicles [19]. Nanomaterials and nanotechnologies have attracted much interest worldwide due to their enormous advantages in biomedicine [20–22] and have been developed as potential delivery vehicles for many biomolecules, including peptides [23–25], proteins [26, 27], RNAs [28, 29], and DNAs [30, 31]. The applications of nanotechnology in drug delivery field can improve the pharmacokinetics and biodistribution of drugs [32], protect them from degradation or rapid clearance [33, 34], and reduce their adverse effects [35]. More than 50 medical products based on nanoparticles have reached the market [36]. Similar to those found in cancer [37], infection-induced inflammation causes vascular abnormalities, including angiogenesis and high vascular density, which contributes to passive accumulation and spread of nanoparticles in deep infectious tissues through the enhanced permeation and retention effect [38]. Herein, we will mainly discuss the current development of nanoparticles for the delivery of AMPs to improve the treatment of deep infections ( Table 1 ).
Summary of Nanoparticles for the Delivery of AMPs
System | AMP | Animal model | References |
---|---|---|---|
Liposomes | PMB | Pseudomonas lung infection | [42] |
PEGylated phospholipid | Aurein-derived AMPs | Murine cutaneous abscess model | [43] |
PLGA modified with poly(vinyl alcohol) | Frog-skin derivative AMPs | Pseudomonas lung infection | [44] |
Dextran nanoparticles | SET-M33 | Pseudomonas lung infection | [46] |
pH-sensitive polyion nanocomposite (CS-DA) | PMB | Pseudomonas lung infection | [47] |
Polymer–peptide conjugate | KLAK and GPLGVRGC | S. aureus subcutaneous infection model | [53] |
AMP derivative | KLVFF | S. aureus muscle infection model | [54] |
Nanoparticles for the delivery of AMPs
Delivery systems approved by the Food and Drug Administration such as liposomes and poly(lactic-co-glycolic acid) (PLGA) nanoparticles are optimum choices, since they have been approved for use in clinic [39, 40]. Liposomes can encapsulate both hydrophilic and lipophilic molecules including AMPs [41]. He et al. encapsulated polymyxin B (PMB) in liposomes comprising 1,2-dipalmitoyl-sn-glycero-3-phosphocholine and cholesterol [42]. The treatment of liposomal PMB showed a 2-fold lower bacterial burden in lung and longer survival time than free PMB in a pneumonia model. In another study, PEGylated phospholipid (DSPE-PEG2000) was employed to deliver a series of aurein-derived AMPs, peptide 73, D-73, RI-73, and 73c ( Figure 1 ) [43]. The nanocomplex of AMPs and DSPE-PEG2000 showed reduced hemolytic activity and superior antibacterial activity, reducing abscess sizes by more than 60% and lowering bacterial loads up to more than 9-fold over free peptide. Casciaro et al. encapsulated a frog-skin derivative AMP, namely Esc peptides (GIFSKLAGKKIKNLLISGLKG-NH2), in PLGA nanoparticles, which were stabilized with poly(vinyl alcohol) ( Figure 2 ) [44]. As compared with free peptide, Esc peptide-loaded nanoparticles exhibited 2- and 1-fold more penetration through the artificial mucus and simulated biofilm, respectively, and effectively reduced the bacterial loads by more than 4 times in acute lung infection caused by Pseudomonas aeruginosa (P. aeruginosa).
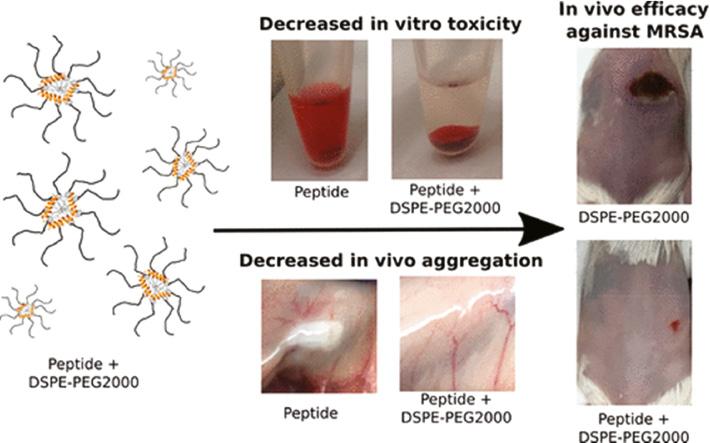
PEGylated phospholipid (DSPE-PEG2000) for the effective delivery of AMPs. The nanocomplex of DSPE-PEG2000 and AMPs showed a low hemolytic activity and high antibacterial efficacy against MRSA in vivo [43]. Reproduced with permission from ACS Infectious Diseases, 2018.
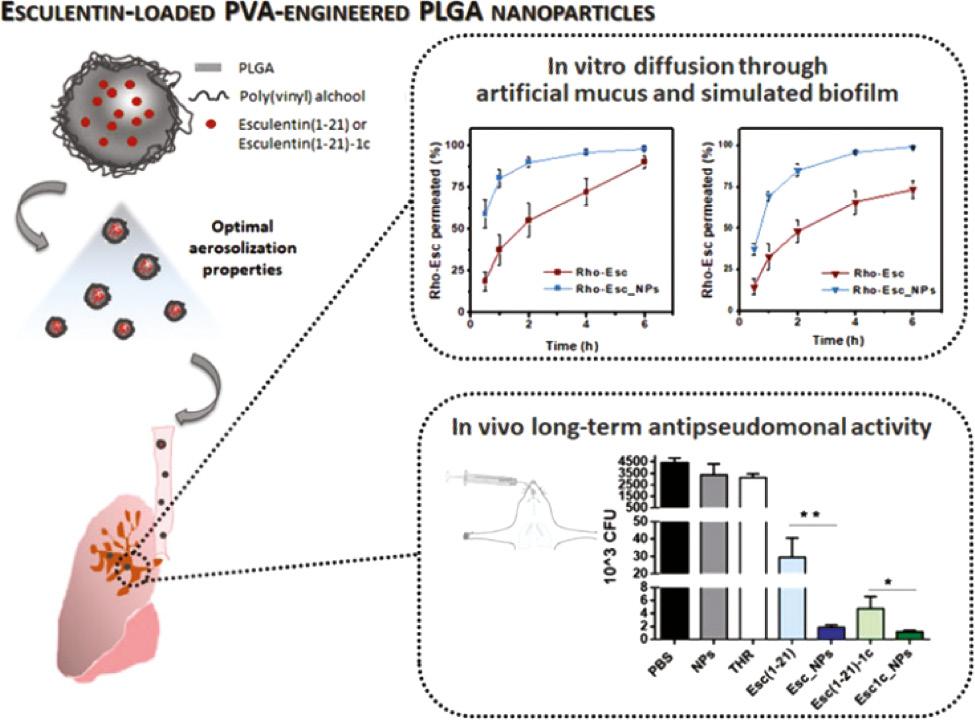
PLGA nanoparticles for the delivery of Esc-derived AMPs. Esc peptide-loaded NPs exhibited more penetration through the artificial mucus and simulated biofilm and effectively reduced the bacterial loads than free peptide [44]. Reproduced with permission from Biomacromolecules, 2019.
Because of the cationic feature of AMPs, anionic nanoparticles have been developed to encapsulate AMPs through electrostatic interaction [24, 45]. Chiara et al. developed a nanosystem (M33-NS) comprising negatively charged dextran nanoparticles and cationic antimicrobial peptide SET-M33 ((KKIRVRLSA)4K2KβA-OH) [46]. M33-NS showed 12-fold more residence time in lung as compared with free peptide, thus improving antimicrobial efficacy against P. aeruginosa in pneumonia model.
Responsive nanoparticles for the delivery of AMPs
In addition, researchers attempt to selectively deliver and release AMPs by designing infection-responsive nanoparticles [47]. When these nanoparticles reach infected sites, AMPs can be selectively released/activated to inhibit bacterial growth. It has been reported that bacterial infections lead to a decrease of pH as well as an increase of enzymes and reactive oxygen species, forming a special microenvironment [48]. For instance, in consideration of the acidic environment of bacterial infection, Chai et al. designed a pH-sensitive charge reversable polyion nanocomposite (CS-DA) for the delivery of PMB ( Figure 3 ) [47]. CS-DA was comprised of chitooligosaccharides (CS) grafted with 2,3-dimethyl maleic anhydride (DA). The amide bonds, generated from the reaction of DA and amino groups of CS, could be hydrolyzed under acidic microenvironment of bacterial infection. CS-DA could effectively encapsulate PMB through electrostatic interaction in physiological condition. In the acidic infectious environment, the amide bonds were cleaved and converted into positively charged amines, leading to a release of PMB. This design not merely maintained the original excellent bactericidal performance of PMB but also significantly decreased the side effects of PMB such as nephrotoxicity. Acid-cleavable groups can also be used to modify AMPs as well as AMPs analogues except for modifying delivery systems. Ye et al. reported a pH-responsive polymer–drug conjugate (PDC) to fight with antimicrobial-resistant (AMR) pathogens ( Figure 4 ) [49]. The PDC was composed of a polymer Hex-Cys-DET, which was modified with acid-responsive DA groups, and conjugated with streptomycin through a pH-sensitive imine bond. PDC was electrically neutral in the bloodstream due to the modification of DA, showing low toxicity in normal tissues, whereas in the acidic infectious site, PDC transformed to a cationic polymer after cleavage of DA; meanwhile, streptomycin was released, showing a synergistic effect to kill bacteria. The PDC showed higher antimicrobial activity than free streptomycin, causing an average of three orders of reductions of bacteria in three infection models. In a P. aeruginosa-infected lung model, all mice treated with PDC survived, whereas the survival rate of mice was only 40% for free streptomycin group after 4 days. Furthermore, PDC treatment caused negligible toxicity in the major organs.
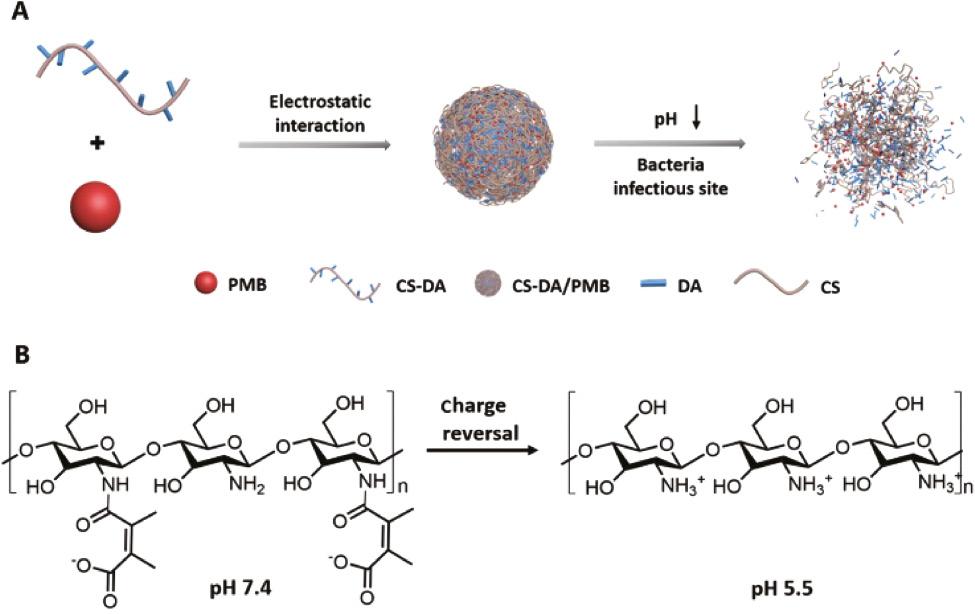
(A) Composition of CS-DA/PMB nanocomplexes. (B) Charge reversal of CS-DA [47]. Reproduced with permission from Advanced Healthcare Materials, 2020.
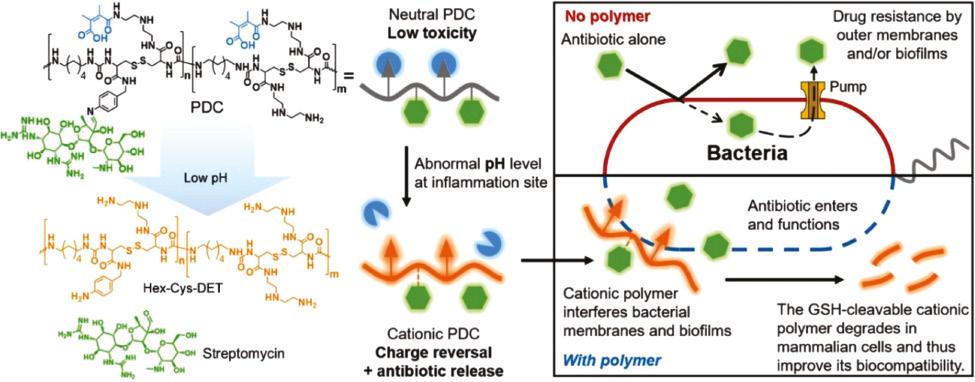
Antibacterial process of the pH-sensitive PDC. The PDC was composed of a polymer Hex-Cys-DET modified with acid-responsive DA groups and conjugated with streptomycin through a pH-sensitive imine bond. In the acidic infectious condition, Hex-Cys-DET transformed to a cationic polymer and streptomycin was released, showing a synergistic effect in reducing bacteria [49]. Reproduced with permission from Advanced Functional Materials, 2020.
Many enzymes overexpresses in the microenvironment of bacterial infection, such as phospholipase [50], protease lipase [51], and gelatinases [52]. Since they could accelerate the hydrolysis of specific bonds, they inspire researchers to design enzyme-responsive delivery system. Wang et al. developed a polymer–peptide conjugate CPC-1, consisting of a chitosan backbone, an AMP (KLAK), and an enzyme-cleavable peptide (GPL-GVRGC) with a PEG ( Figure 5 ) [53]. CPC-1 assembled into PEGylated nanoparticles, which shielded AMPs and significantly reduced the toxicity of AMPs. CPC-1 showed a significantly decreased hemolytic activity against red blood cells (9.4%) in respect to KLAK treatment (94.3%). Once it reached infectious site, the enzyme-cleavable peptide was cleaved by gelatinase to peel off the protective PEG layer, which led to a transformation of nanoparticles into fibrous nanostructures and exposed AMP (KLAL) to kill bacteria. Interestingly, the in situ morphological transformation significantly increased the accumulation and retention of AMPs in the infected area. CPC-1 showed a long half-life in vivo up to 4 days and high antibacterial activity with a 10-fold decrease of bacterial colony as compared with CPC-2, a control nanoparticle with non-cleavable peptide.
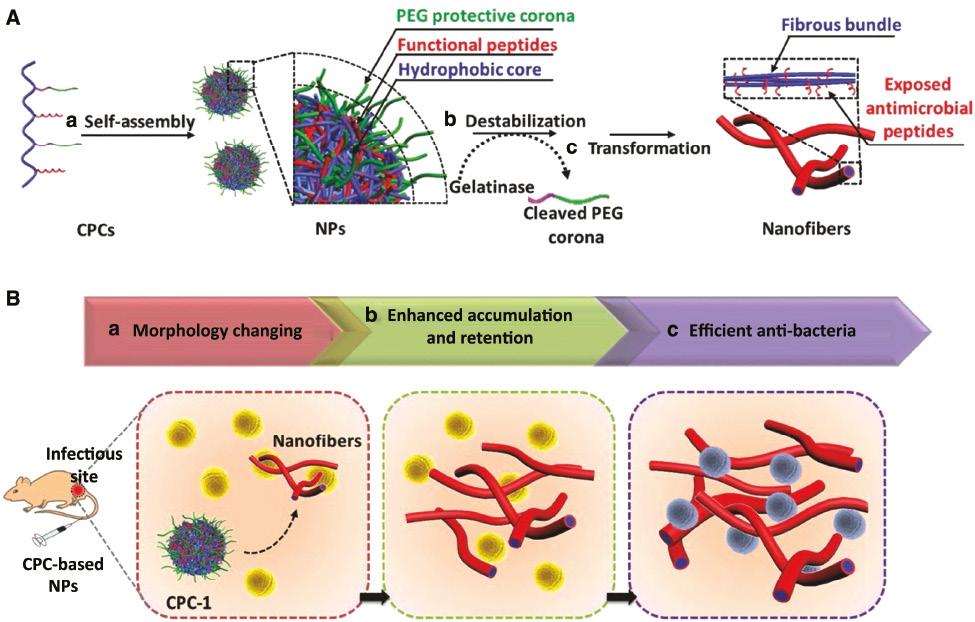
(A) Self-assembly and enzyme-responsive morphology transformation of chitosan–peptide conjugates (CPCs). (a) CPC-1 self-assembled into PEGylated nanoparticles (NPs); (b) bacterial gelatinase cleaved the enzyme-responsive peptide to strip off the protective corona; (c) CPCs transformed into fibrous structures. (B) In situ morphology transformation of CPC to improve the antibacterial efficacy in the infectious sites [53]. Reproduced with permission from Advanced Materials, 2017.
Nanomaterials not only can be designed to target infectious tissue but also can specifically recognize and kill bacteria. Wang et al. designed a human defensin-6 mimic peptide (HDMP), which was constitutive of a ligand peptide (RLYLRIGRR), a β-sheet peptide (KLVFF), and bis-pyrenes ( Figure 6 ) [54]. HDMP self-assembled into sphere nanoparticles with minimal toxicity against normal tissues/cells. At the infection sites, the ligand peptide recognized and specifically bound to lipoteichoic acid on the Gram-positive bacteria to induce a transformation of HDMP nanoparticle into nanofibers. HDMP (5 mg/kg) showed a superior therapeutic effect than vancomycin (5 mg/kg) on the MRSA (methicillin-resistant Staphylococcus aureus)–infected mouse model. All the mice treated by HDMP were alive, whereas the survival rate of the vancomycin group was 83.3% in 96 h.
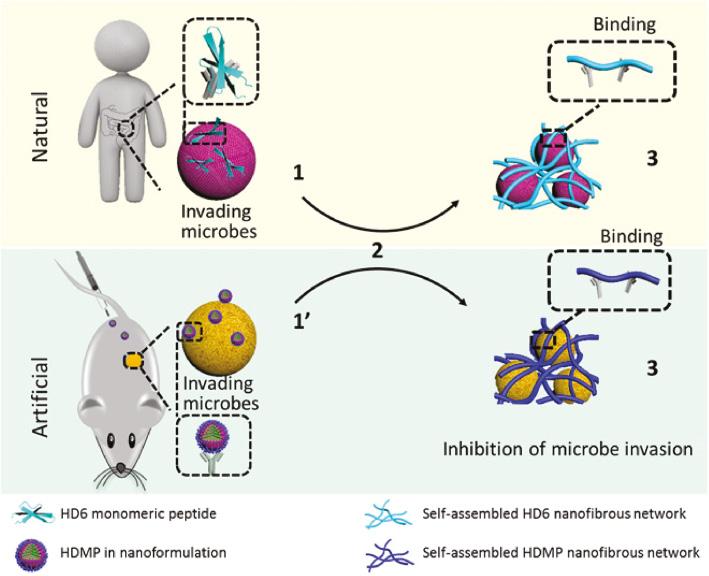
Antimicrobial process of natural human defensin 6 (HD6) and artificial HD6 mimic peptide (HDMP). The HDMP imitates the HD6 that (2) self-assembles when it encounters microbes, thus (3) forming fibrous networks for capturing invading bacteria [54]. Reproduced with permission from Science Advances, 2020.
Conclusion
Nanoparticle delivery systems have shown great potential for the delivery of AMPs to combat drug-resistant bacteria, particularly in deep organ infections. This strategy provides many advantages over free peptides: it reduces toxicity to normal tissues; it improves the pharmacokinetics of AMPs; it can achieve superior distribution of AMPs in the infection tissues with enhanced bioavailability; it can be designed as intelligent nanocarriers for target infectious tissues and bacteria. In the future, the development goal of the delivery systems of AMPs is to design AMPs delivery systems to achieve selective activation and active target in the infectious sites, thus improving the therapeutic efficacy against bacterial infection and reducing the toxicity against normal tissues.